 |
|
|
Contained fluids heated from below
spontaneously organize into convection cells when sufficiently
far from conductive equilibrium. Fluids can also be organized
by surface tension, and other forces and structures at the
top, and by imposed horizontal temperature gradients and
motions. At mantle conditions rocks are generally treated
as fluids.
Plate tectonics was once regarded as
passive motion of plates on top of mantle convection cells
but it now appears that continents and plate tectonics organize
the flow in the mantle and that the mantle is the passive
element. The flow is driven by instability of the cold surface
layer and near-surface lateral temperature gradients such
as imposed by slabs and continents. Plate tectonics may
be a self-driven, far-from-equilibrium system that organizes
itself by dissipation (entropy production) in and between
the plates. The mantle may simply be a provider of energy
and material.
The effect of pressure suppresses the
role of the lower thermal boundary layer (TBL) at the core-mantle-boundary
(CMB) interface. The state of stress in the lithosphere
defines the plates, plate boundaries and locations of midplate
volcanism. Fluctuations in stress, due to changing boundary
conditions, are responsible for global plate reorganizations
and evolution of volcanic chains. In Rayleigh-Benard convection,
by contrast, temperature fluctuations are the important
parameter. In plume theory, plates break where heated or
uplifted by plumes. Ironically, the fluid flows in the experiments
by Benard, which motivated the theory by Rayleigh, were
driven by surface tension, i.e. stresses at the surface. |
|
|
Mantle convection is quite different from the usual
pot-on-a-stove metaphor. A large bowl of several superposed fluids and
ice cubes in a microwave oven, programmed to decrease the power with
time, would be a better, but still incomplete, analogy. The missing
element in laboratory and kitchen experiments, and most computer simulations,
is pressure. The mantle is heated from within, cooled from above and
cools off with time (secular cooling). All of these effects drive convective
motions. The distribution of radioactive elements within the Earth is
not uniform. Heating from below is minor, at least for the mantle as
a whole. Viscosity varies with depth and temperature. Solid-solid phase
changes (some exothermic and some endothermic), occur at various depths.
Rheology changes with stress.
There are text books, monographs and hundreds of papers
on the subject of thermal convection but few are applicable to the mantle.
Mantle convection is far from being a closed subject. Computer simulations
have not yet included a self-consistent thermodynamic treatment of the
effect of temperature, pressure and volume on the physical and thermal
properties, and understanding of the “exterior” problem
(the surface boundary condition) is in its infancy. Plate tectonics
itself is implicated in the surface boundary condition. Melting is an
important aspect of real mantle convection. Sphericity, pressure and
the distribution of radioactivity break the symmetry of the problem
and the top and bottom boundary conditions play quite different roles
than in the simple calculations and cartoons of mantle dynamics and
geochemical reservoirs. Conventional (Rayleigh-Benard) convection theory
may have little to do with plate tectonics. The research opportunities
are enormous.
|
The history of ideas
Convection can be driven by bottom heating, top or side cooling, and
by motions of the boundaries. Although the role of the surface boundary
layer and “slab-pull” are now well understood and the latter
is generally accepted as the prime mover in plate tectonics, there is
a widespread perception that active hot upwellings from deep in the
interior of the planet, independent of plate tectonics, are responsible
for “extraordinary” events such as plate reorganization,
continental break-up, extensive magmatism and events far away from current
plate boundaries. Plate tectonics is considered by some to be an incomplete
theory of mantle dynamics. Active upwellings from deep in the mantle
are viewed as controlling some aspects of surface tectonics and volcanism,
including reorganization, implying that the mantle is not passive. These
have been modeled by the injection of hot fluids into the base of a
tank of motionless fluid. This is called the plume mode of mantle convection.
Numerical experiments show that mantle convection is controlled from
the top by continents, cooling lithosphere, slabs and plate motions
and that plates not only drive and break themselves but can control
and reverse convection in the mantle (1-6). Supercontinents and other
large plates generate spatial and temporal temperature variations. The
migration of continents, ridges and trenches cause a constantly changing
surface boundary condition, and the underlying mantle responds passively
(7-10). Plates break up and move, and trenches roll back because of
forces on the plates and interactions of the lithosphere with
the mantle. Density variations in the mantle are, by and large, generated
by plate tectonics itself, for example through slab cooling, refertization
of the mantle, continental insulation, and these also affect the forces
on the plates. Surface plates are constantly evolving and reorganizing
although major reorganizations are infrequent. They are mainly under
lateral compression although local regions having horizontal least-compressive
axes may be the locus of dikes and volcanic chains.
The mantle is generally considered to convect as a single layer (whole
mantle convection) or, at most two (the standard geochemical model).
However, the mantle is more likely to convect in multiple layers as
a result of gravitational sorting during accretion and the density difference
between the mantle products of differentiation.
|
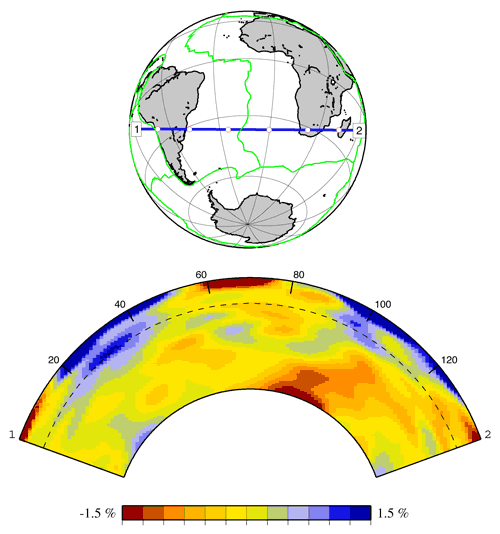
Cross section through the whole-mantle tomography model of Ritsema
et al. (1999) showing the strong heterogeneity that characterises the
upper mantle and the core-mantle boundary region, contrasting with the
weak heterogeneity in the mid-mantle. The mantle may be divided into
three or more chemically distinct layers.
|
Boundary Conditions
The core has low viscosity and high thermal conductivity so the base
of the mantle is in contact with a stress-free isothermal bath. The
top boundary condition is plate tectonics. It is not an isothermal,
stress-free, homogeneous, uniform boundary condition. If the plates
are held together by lateral stress (7-10) then the surface must be
free to self-organize, a condition not yet allowed in any simulation.
Reorganization means the ability to form new plate boundaries and generate
new plates that are consistent with the ever-changing stress state of
the lithosphere. In the absence of plates or a high viscosity lid the
mantle would experience Rayleigh-Benard convection. Above a critical
Rayleigh number fluids spontaneously convect and self-organize. Buoyancy
of the fluid, which is dependent on the coefficient of thermal expansion
(expansivity) and temperature fluctuations, drives the flow and the
viscosity forces of the fluid dissipate the energy. Temperature-dependent
viscosity, a semi-rigid lithosphere (held together by lateral compressive
stresses) and buoyant continents (and thick crust regions) completely
change this. Gravitational forces on cooling plates cause them to move.
Dissipation takes place in and between the plates, causing them to self-organize
and to organize the underlying weaker mantle.
Plate tectonics, to a large extent, is driven by the unstable surface
TBL and therefore resembles convection in fluids which are cooled from
above (see http://anquetil.colorado.edu/VE/convection.shtml).
Pressure decreases the expansivity and Rayleigh number so it is difficult
to generate buoyancy or vigorous small-scale convection at the base
of the mantle. In addition, heat flow across the CMB is about an order
of magnitude less than at the surface so it takes a long time to build
up buoyancy. In contrast to the upper TBL, which involves frequent ejections
of narrow dense slabs into the interior, the lower TBL is sluggish and
does not play an active role in mantle convection. CMB upwellings are
expected to be thousands of kilometers in extent and embedded in high-viscosity
mantle.
Lithospheric architecture and slabs set up lateral temperature gradients
that drive small-scale convection. For example, a newly opening ocean
basin juxtaposes cold cratonic temperatures of about 1000°C at 100
km depth with asthenospheric temperatures of about 1400°C. This
lateral temperature difference sets up convection. Convective flows
driven by this mechanism can reach speeds of 15 cm/yr and may explain
volcanism at the margins of continents and cratons, and at oceanic
and continental rifts. Shallow upwellings resulting from this
mechanism are intrinsically three-dimensional and plume-like.
|
Fundamentals
Materials usually expand when heated. This causes them
to rise when embedded in compositionally similar material. Pressure drives
atoms closer together and suppresses the ability of high temperatures
to create buoyancy. This is unimportant in the laboratory but it also
means that laboratory simulations of mantle convection, including theinjection
experiments used to generate plumes, are not relevant to the mantle. Unfortunately,
computer simulations are generally used to confirm the laboratory results
and, when applied to the mantle, also ignore the effects of pressure on
material properties. In fact, the effects of temperature are also generally
ignored except the effect of temperature on density. This is called the
Boussinesq approximation. This works fine in the laboratory, but does
not apply to the mantle.
Lateral variations in temperature are what drives thermal convection.
Lateral variations in pressure are generally unimportant since the pressure
in the material outside of the rising element is about the same as inside.
But the increase in pressure with depth means that viscosity, thermal
conductivity and expansivity change, making it harder for material to
convect. The system responds by increasing the dimensions of the thermal
instabilities in order to maintain buoyancy and to overcome viscous resistance.
A closed or isolated system at equilibrium returns to equilibrium if
perturbed. In a tank of fluid, or the mantle, with a cold surface and
a hot bottom, heat will flow by conduction alone unless the temperature
gradient gets too large. The stable conduction situation is called equilibrium.
A far-from-equilibrium dissipative system, provided with a steady source
of energy or matter from the outside world, can organize itself via its
own dissipation. It is sensitive to small internal fluctuations and prone
to massive reorganization. The fluid in a pot heated on a stove evolves
rapidly through a series of transitions with complex pattern formation
even if the heating is spatially uniform and slowly varying in time. The
stove is the outside source of energy and the fluid provides the buoyancy
and the dissipation (via viscosity). Far-from-equilibrium self-organization
and reorganization require an open system, a large, steady, outside source
of matter or energy, non-linear interconnectedness of system components,
dissipation, and a mechanism for exporting entropy products. Under these
conditions the system responds as a whole, and in such a way as to minimize
entropy production (dissipation). Certain fluctuations are amplified and
stabilized by exchange of energy with the outside world. Structures appear
which have different time and spatial scales than the energy input).
Similar considerations apply to a fluid cooled from above. The cold surface
layer organizes the flow and drives the convection. If the fluid has a
strongly temperature-dependent viscosity, or if buoyant things are floating
on top, only part of the surface layer is able to circulate into the interior.
If the upper mantle is near or above the melting point there are other
sources of buoyancy and dissipation and the possibility of lubrication.
Volcanic chains can form as a result of buoyant dikes breaking through
the surface layer at regions of relative extension. Melts are predicted
to pond beneath regions of lateral compression. |
Plume Type Convection
The idea that a deep TBL may be responsible for narrow structures such
as volcanic chains is based on heating-from-below experiments and calculations,
or injection experiments. The effects of pressure on thermal properties
are not considered (the Boussinesq approximation). In the Earth the
effects of temperature and pressure on convection parameters cannot
be ignored and these must be determined as part of the solution in a
self-consistent way. Such calculations, and mantle tomography, suggest
that it is highly probable that the mantle below 2,000 km depth, and
possibly below 1,000 km depth is isolated from the surface, except by
Newton’s and Fourier’s laws. Furthermore, it is the cooling
of the mantle that controls the rate of heat loss from the core. The
core does not play an active role in mantle convection. The magnitude
of the bottom TBL depends on the cooling rate of the mantle, the pressure
and temperature dependence of the physical properties and the radioactivity
of the deep mantle. The local Rayleigh number of the deep mantle is
very low.
|
Layered
mantle convection
Chemical boundaries are hard to detect by seismic techniques
but the evidence favors one such boundary near 1,000
km. The seismic boundary at an average depth of 650
km is primarily due to a solid-solid phase change, in
mantle minerals, with a negative Clapeyron slope. Slabs
can be halted at such a boundary but if they accumulate
they can punch through.
Observations also suggest the presence of one or more
chemically distinct layers at the base of the mantle that may extend,
in places, as high as 1,000 km from the CMB. This region exhibits large-scale
sluggish behavior as appropriate for high Prandt number, low Rayleigh
number convection. This kind of chemical and gravitational stratification
resolves various geodynamic and geochemical paradoxes and is more consistent
with petrology and mineral physics than the one- and two-layer models
that are most discussed in the literature.
It is generally believed that geochemical observations
support models of layered mantle convection and the popular geochemical
box models. However, it is chemical heterogeneity that is demonstrated
by the data, which cannot determine the depth, size or configurations
of the inhomogeneities. Isolated deeper layers that do not currently participate
in surface volcanism are sometimes called “hidden”,“stealth”
or “phantom” reservoirs. They show up as “missing components”
and “geochemical paradoxes”.
If pressure is ignored it requires about a 6% increase
in intrinsic density for a deep mantle layer to be stable against a temperature-induced
overturn. Plausible differences in density between most mantle products
of accretional differentiation intermediate in density between the crust
and the core are about 1 or 2%, if the variations are due to changes in
silicon, aluminum, calcium and magnesium. Changes in iron content can
give larger variations. Such density differences have been thought too
small to stabilize stratification. However, when pressure is taken into
account such slightly denser layers become trapped, although their effect
on seismic velocities can be slight. These are therefore “stealth”
layers or reservoirs which are below the ability of seismic waves to detect,
except by special techniques. Multilayered convection simulations are
avoided for the reason that modelers think there is no evidence for it,
and the calculations are difficult and time consuming. This is a major
hindrance for advances in mantle dynamics and geochemistry and an opportunity
for future research.
A chemically stratified mantle with variable-depth chemical
boundaries near 1,000 and 2,000 km and a lower mantle depleted in radioactive
elements appears to satisfy available geochemical and geophysical constraints. |
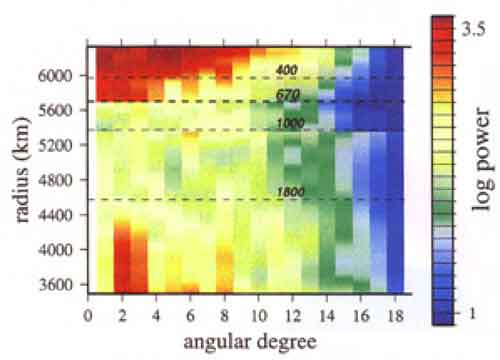
Spherical harmonic power spectrum of velocity throughout the mantle
(from Gu et al., 2001). The Earth is clearly characterised by strong
heterogeneity in the longer-wavelength components in the lithosphere
and upper mantle and the lowermost mantle, with little heterogeneity
in between. The three major regions of the mantle are evident. There
may also be chemically distinct layers at the very base of the mantle
(D") and a buoyant, refractory layer at the top of the mantle (the
perisphere).
|
The forces
on plates
The creation of new plates at ridges, the subsequent
cooling of these plates, and their ultimate subduction at trenches introduce
forces which drive and break up the plates. They also introduce chemical
and thermal inhomogeneities into the mantle. Plate forces such as ridge
push, slab pull, and trench suction are basically gravitational forces
generated by cooling plates. They are resisted by transform fault, bending
and tearing resistance, mantle viscosity and bottom drag. If convection
currents dragged plates around, the bottom drag force would be the most
important. However, there is no evidence that this is a strong force,
and even its sign is unknown (driving or resisting drag). The thermal
and density variations introduced into the mantle by subduction also generate
forces on the plates.
The deep mantle, even if convectively isolated from the
upper mantle, affects the elevation and stress state of the lithosphere.
Density variations in the mantle also cause variations in elevation and
stress in the plate, which are added to the plate forces. Hot regions
of the lower mantle will heat the upper mantle and may control, to some
extent, the locations of supercontinents and long-lived subduction zones.
Even if the mantle is irreversibly chemically stratified into two or more
layers, the deep layers will have an effect on geophysical observables.
Regions of high elevation, tensile stress and volcanism will tend to be
above low-density regions of the deep mantle unless counteracted by gravitational
forces in the plates themselves and at plate boundaries.
Because of the high viscosity of the deep mantle the
warm regions are semi-permanent compared to features in the upper mantle.
The large viscosity contrast means that the various layers are more likely,
on average, to be thermally coupled than shear coupled. From a tomographic
point of view, this means that some mantle structures may appear to be
continuous even if the mantle is stratified (see (11) and http://karel.troja.mff.cuni.cz/staff/HANKA_CIZKOVA/Anim/animace.htm). |
The core-mantle
boundary region
The TBL at the base of the mantle generates a potentially unstable situation.
The effects of pressure increase the thermal conductivity, decrease the
thermal expansion and increase the viscosity. This means that conductive
heat transfer from below is more efficient than at the surface, that temperature
increases have little effect on density and that any convection will be
sluggish. Although the amount of heat coming out of the core may be appreciable,
it is certainly less (~10%) than that flowing through the surface. The
net result is that lower-mantle upwellings take a long time to develop
and they must be very large in order to accumulate enough buoyancy to
overcome viscous resistance. The spatial and temporal scale of core-mantle-boundary
instabilities are orders of magnitude larger than those at the surface.
This physics is not captured in laboratory simulations or calculations
that adopt the popular Boussinesq approximation. Pressure also makes it
easier to irreversibly chemically stratify the mantle. A small intrinsic
density difference due to subtle changes in chemistry can keep a deep
layer trapped since it requires such large temperature increases to make
it buoyant. Layered mantle convection is the likely outcome. |
A
Primer on Convection
A
system cooled from above or heated from within will develop
an upper thermal boundary layer which drives the system.
The
thermal boundary layer (plate, slab) is the only active element.
All
upwellings are passive, and diffuse.
For
large Prandtl number (the mantle) the mechanical boundary
layers are the size of the mantle.
The
scale of thermal boundary layers (plate thickness) is controlled
by the Rayleigh number (Ra), which for the top
is of the order of hundreds of km.
Ra
is controlled both by physical properties (conductivity, expansivity
etc.) and environment (heat flow, temperature gradients etc.).
Both
these factors cause Ra to be orders of magnitude
lower at the base of mantle than at top. Therefore convective
vigor is orders of magnitude less at the base of mantle.
The
mechanical and thermal boundary layers at the base of mantle
are therefore of the order of thousands of kilometers in lateral
dimensions.
Radioactivity
does not contribute to unstable superadiabatic gradients because
the time constants are much greater than convective time constants. |
|
|
Notes
In the Morgan
plume theory, upwellings are the active narrow elements
and downwellings are passive and diffuse. This is
the exact opposite of cooled-from-above-systems and
what the geophysical data tell us. Morgan plume theory
holds for systems heated from below and not cooled
from above.
If there are no
pressure effects and if all the heat enters from below
and leaves from the top then there is symmetry and
both upper and lower TBL are active.
Sphericity, pressure,
continents and distribution of radioactivity break
symmetry and mean that lower TBL instabilities are
sluggish and gigantic compared with upper TBL instabilities
(slabs).
Continents/cratons
also contribute to the lateral thermal gradients that
drive convection from the top.
It is lateral thermal
gradients, not vertical ones, that drive convection.
The lithosphere,
instead of the mantle, controls cooling of mantle.
|
- M. Gurnis, Nature, 332, 695 (1988).
- M. Gurnis, S. Zhong, Geophys. Res. Lett., 18,
581 (1991).
- M. Gurnis, S. Zhong, J. Toth, The history and dynamics of global plate
motions, AGU, Geophysical Monograph 121, pp. 73, (2000)
- J. P. Lowman, G. T. Jarvis, Geophys. Res. Lett., 20,
2087 (1993).
- J. P. Lowman, G. T. Jarvis, J. Geophys. Res., 104,
12,733 (1999).
- S. Zhong, M. Gurnis, Geophys. Res. Lett., 22,
981 (1995)
- Anderson, D. L., Top-down tectonics,
Science, 293, 2016 (2001).
- Anderson, D. L., A statistical test of the two reservoir model for
helium, Earth Planet. Sci. Lett., 193, 77
(2001).
- Anderson, D. L., 2001, How many Plates?, Geology, 30,
411 (2002).
- Anderson, D. L. Plate Tectonics as a Far- From- Equilibrium Self-Organized
System, in Plate Boundary Zones, ed. S. Stein, AGU Monograph,
(2002).
- Cizkova, H., Cadek, O., van den Berg, A.P. and N.J. Vlaar, Can
lower mantle slab-like seismic anomalies be explained by thermal coupling
between the upper and lower mantles? Geophys. Res. Lett.,
26, 1501-1504, 1999.
General References
- Agee, C. B. and Walker, D., Mass balance and phase density constraints
on early differentiation of chondritic mantle, Earth Planet. Sci.
Lett., 90, 144 (1988).
- Anderson,
D. L., Theory of the Earth, Blackwell Scientific Publications,
Boston, pp. 366 (1989). [Chapter 8 is relevant to irreversible stratification
of mantle and low U in the lower mantle.]
- Anderson, D. L., Where on Earth is the Crust?, Physics Today,
March 1989, 38-46. (1989).
- Clark, S. P., and Turekian, K. K., Thermal constraints on the distribution
of long-lived radioactive elements in the Earth: Phil. Trans. R.
Soc. Lond., 291, 269-275 (1979).
- Coltice, N., and Ricard, Y., Geochemical observations and one layer
mantle convection: Earth Planet. Sci. Lett., 174,
125-137 (1999).
- Conrad, C. P., and Hager, B. H., Mantle convection with strong subduction
zones: Geophys. J. Int., 144, 271-288 (2001).
- Cordery, M. J., Davies, G. F., and Campbell, I. H., Genesis of flood
basalts from eclogite-bearing mantle plumes: J. Geophys. Res.,
102, 20,179-20,197 (1997).
- Cserepes, L., Yuen, D. A., and Schroeder, B. A., Effect of the mid-mantle
viscosity and phase-transition structure of 3D mantle convection: Phys.
Earth. Planet. Int., 118, 135-148 (2000)
- Davaille A., Simultaneous generation of hotspots and superswells
by convection in a heterogeneous planetary mantle: Nature,
402, 756-760 (1999).
- Davies, G. F., Dynamic Earth: Plates, Plumes and Mantle Convection:
Cambridge University Press, Cambridge, 458 pp. (2000).
- Gu, Y., A.M. Dziewonski, S. Weijia, and G. Ekstrom, Models of the
mantle shear velocity and discontinuities in the pattern of lateral
heterogeneities, J. geophys. Res., 106, 11,169-11,199
(2001).
- King, S. D., and Anderson, D. L., An alternative mechanism of flood
basalt formation: Earth Planet. Sci. Lett., 136,
269-279 (1995).
- Ritsema, J., H.J. van Heijst, and J.H. Woodhouse, Complex shear wave
velocity structure imaged beneath Africa and Iceland, Science,
286, 1925-1928 (1999).
- Schubert, G., Turcotte, D., Olson, P., Mantle convection in the Earth
and planets: C. U. Press, 956 pp. (2001).
- Scrivner, C. and Anderson, D. L., The effect of post Pangea subduction
on global mantle tomography and convection: Geophys. Res. Lett.,
19, 1053-1056 (1992).
- Tackley, P. J., Mantle convection and plate tectonics: Toward an integrated
physical and chemical theory: Science, 288,
2002-2007 (2000).
- Tackley, P., Three dimensional simulations of mantle convection with
a thermo-chemical basal boundary layer: in: M. Gurnis, M. et al., eds.,
The Core-Mantle Boundary Region, Washington, AGU, 334 pp. (1998).
- Turcotte, D.L. and G. Schubert, in Geodynamics, John Wiley
& Sons, New York, 450 pp. (1982).
- Wen, L. and Anderson, D. L., Layered mantle convection: A model for
geoid and topography: Earth Planet. Sci. Lett., 146,
367-377 (1997).
- Wen, L. and Anderson, D. L., Slabs, hotspots, cratons and mantle
convection revealed from residual seismic tomography in the upper mantle:
Phys. Earth Planet. Int., 99, 131-143 (1997).
- http://jspc-www.colorado.edu/~szhong/mantle.html
|
|
|