 |
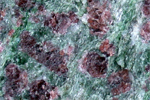 |
The layered mantle revisited
An eclogite
reservoir
|
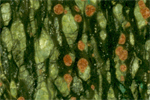 |
|
|
Summary
Mass balance
calculations suggest that the mantle as a whole
contains about 7% basalt/eclogite (Anderson, 1989a
Theory of the Earth, Chapter 8; Rudnick et al.,
1998). Where and how is this sequestered? If the
basalt/eclogite is distributed throughout the
upper mantle then the average composition of this
region of the mantle may approach pyrolite in
composition. However, it is also plausible that
the upper mantle and transition zone (TZ) are
petrologically and density stratified, with the
buoyant material (harzburgite, depleted peridotite)
concentrated at shallow levels in a “perisphere”,
and the denser material (eclogite, garnetite)
concentrated in the TZ or above. The feasibility
of this depends on the density and composition
of the eclogite (particularly the SiO2-CaO-SiO2-contents)
and the density and composition of the lower mantle.
Eclogite-rich layers would be intermittent because
of low melting points and the temperature-dependency
of the relevant phase changes.
I suggest that
under certain circumstances the lower crust transforms
to dense eclogite and delaminates. It then sinks
to the level of neutral buoyancy, which can be
anywhere from about 300 to 650 km depth. There,
eclogite will comprise a low-velocity layer. Thus,
dense, fertile sinkers or neutrally buoyant cold
eclogite layers may be mistaken in tomographic
images for hot plumes.
|
Introduction
A variety of evidence suggests that
about 7% of the mantle may be eclogite. The question
is, how is it distributed and where is it stored? Ringwood
et al. (1994) and Hirose et al. (1999)
argue that recycled oceanic crust eventually sinks into
the lower mantle. Anderson (1979, 1987, 1989a,b)
argued that it is trapped in the TZ, in the depth interval
410 – 650 km, which comprises about 11% of the
mantle. The depth of trapping depends on the compositions
and densities adopted for the eclogite and the adjacent
mantle, and the composition of the lower mantle. If
one adopts MORB as the protolith of eclogite it will
be particularly dense because of its high SiO2 (stishovite)
content compared to other mafic protoliths, such as
cumulates, restites, delaminated continental crust and
the average composition of oceanic crust. On the other
hand pyrolite is also not a completely satisfactory
model for the TZ and the lower mantle (Cammarano
et al., 2005; Duffy & Anderson, 1989,
Lee et al., 2004). The density of eclogite
relative to pyrolite may not be a pertinent guide to
the fate of eclogite.
Long-lived reservoirs in the mantle must be either denser
than the overlying layers, or highly viscous blobs,
advected but resisting stretching and mixing. There
are various options for bringing this material to the
surface. If the material has a low melting point, such
as eclogite, it need not be particularly hot, or embedded
in a thermal boundary layer (TBL) in order to become
buoyant. It just needs to heat up to near ambient temperatures
(see Reheated
slabs pages). Because of the effect of pressure
on melting point, a shallow, e.g., upper mantle,
location is favored for such a mechanism to operate.
There is now general agreement there is a subduction
barrier to eclogite between about 650 km (or even shallower)
and 720 km (or even deeper). The window is even broader
for Si-poor eclogites such as cumulates and many non-MORB
protoliths. If the lower mantle is denser than pyrolite
(e.g., Anderson, 1989b; Lee et al.,
2004) then eclogites may be trapped in or above the
TZ until they melt. Recycling and remelting of large
blocks of eclogite is distinct from the thermal plume
hypothesis and distinct from the pyroxenite vein hypothesis
for increasing fertility. Thermal plumes originate in
TBLs and require heating from below, and they are not
an intrinsic part of plate tectonics.
The subduction of oceanic lithosphere
into Earth's interior is thought to drive convection
and create chemical heterogeneity in the mantle. The
delamination of lower continental crust also affects
the dynamics and composition of the mantle. The fates
of basaltic crust, of the underlying peridotite layer,
and of delaminated crust may differ because of differences
in age, temperature, melting temperature, chemistry
and density. It has been suggested that subducted crust
(eclogite), although denser than ambient mantle at shallow
depths, may in fact become buoyant somewhere between
560 km and the 650-km discontinuity, and therefore might
be gravitationally trapped in the TZ to form an eclogite-rich
or garnetite layer (Anderson, 1989b).
What might a chemically stratified
mantle look like? The density and shear-wave seismic
velocity of crustal and mantle minerals and rocks at
standard temperature and pressure are arranged approximately
according to increasing density in Figure 1. This approximates
the situation in an ideally chemically stratified mantle.
P and T effects may change the ordering and the velocity
and density jumps. Eclogite can settle to various levels,
depending on composition; the eclogite bodies that can
sink to greater depths because of their density have
low seismic velocities compared to other rocks with
similar density. Note that MORB-eclogite contains stishovite
at high pressure and may sink below about 500 km. Eclogite
has a much lower melting point than peridotites and
will eventually heat up and rise, even if it does not
lie in a TBL, creating a sort of “yo-yo tectonics”.
The ilmenite form of garnet and enstatite is stable
at low temperature but will convert to more buoyant
phases as it warms up. Velocity decreases do not necessarily
imply hot mantle. Low-velocity zones (LVZs) have been
found by seismology at various depths above 720 km.
Depths of prominent seismic reflectors are noted.
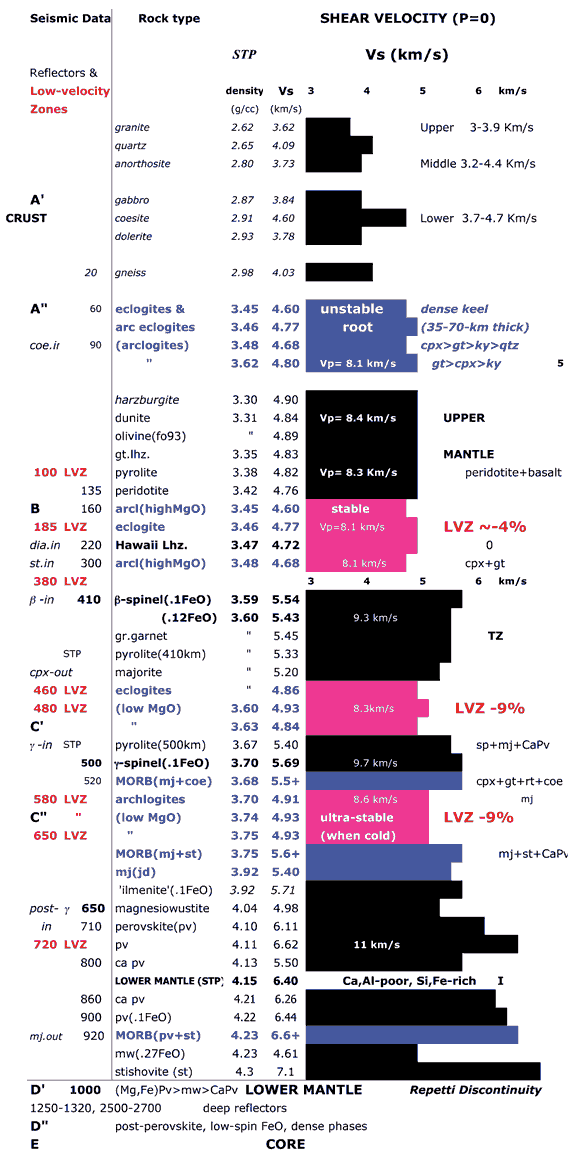
Figure 1: Rocks and minerals arranged
mostly in order of increasing density.
Thermochemical
convection
Two-dimensional simulations of simple
mantle convection suggest that for whole-mantle convection
the mantle should be homogenized in a time-scale of
less than 1 Gyr, although unmixed patches may remain.
The rate at which blobs of inserted material are stretched
and assimilated into the flow depends on their relative
viscosity; viscous blobs may survive intact for many
mantle overturns. All such calculations assume vigorous
convective stirring. If the mantle is layered or if
pressure effects on physical properties are considered,
convection is much less turbulent or chaotic. It is
more probable that subducted and delaminated materials
settle to equilibrium depths without being entrained
into mantle flow. In fact, if mantle flow is mainly
a result of sinking slabs and surface plates, then there
will be little mixing.
Problems with, and alternatives to,
efficient convective mixing are discussed by Meibom
& Anderson (2003). They showed that a spectrum
of basalt types can be generated simply by sampling,
in different ways, a statistically heterogeneous mantle.
Plate tectonic processes and recycling can give this
kind of heterogeneity but can also create a chemically
stratified mantle. Since the products of mantle melting
and differentiation are so disparate in density, they
are likely to settle to different depths. If eclogite,
for example, is denser than upper mantle rocks, but
intrinsically less dense than lower mantle lithologies,
then it would settle into the TZ. However, because of
its complex phase relations with temperature, low melting
point, and possibly high radioactivity, it can change
both its density and relative position over time. Although
eclogite is usually considered to be a dense rock, Figure
1 shows that it can have similar densities to various
peridotite minerals and assemblages that are believed
to make up the mantle between 100 and 400 km. Cold eclogite
may sink as far as the gamma-spinel phase change near
500 km depth. Quartz-rich protoliths such as unaltered
MORB may sink deeper because of the high density of
stishovite (Hirose et al., 1999). Eclogite
is just one example; various peridotites and magmas
differ in intrinsic density by amounts more than can
be overcome by normal temperature variations, and should
therefore concentrate at various levels. Even the crust
and continental lithosphere appear to stratified by
intrinsic density.
Eclogite
in the mantle
Mantle eclogites have a variety of
origins. Eclogites may be produced by high-pressure
crystallization, they may be remnants of subducted oceanic
crust, or the residue of partial melting of such crust.
Some eclogites may be products of metamorphism of mafic
lower continental crust, i.e., gabbroic to anorthositic
protoliths and some may be high-pressure garnet-pyroxene
cumulates or low-pressure plagioclase-pyroxene-olivine
cumulates. High MgO eclogites may represent the lower
parts of subducted oceanic crust or foundered mafic
lower continental crust.
The main minerals in eclogite at low
pressure are clinopyroxene and garnet. Accessory phases
can be rutile, quartz, kyanite and orthopyroxene, depending
on the starting composition. Below about 100 km depth
excess silica converts to coesite, then stishovite,
and the kyanite disappears. At TZ pressures the clinopyroxene
dissolves in garnet, forming majorite; eclogite at the
top of the TZ comprises garnetite+stishovite. At very
high pressures calcium perovskite becomes stable. Hirose
et al. (1999) report the phase relations and melting
temperatures of a moderately differentiated MORB sample
from the north Atlantic at pressures up to 64 GPa (equivalent
to 1,500 km depth). This sample contains stishovite
and Ca-perovskite at pressures greater than 24 GPa (equivalent
to depths greater than 720 km), and is predicted to
become denser than pyrolite at that depth. Stishovite
has a density of 4.3 g/cm3 and shear seismic
velocity of 7.1 km/s, much greater than even the in-situ
values of the mantle down to depths of about 800 km.
Hirose et al. (1999) predict that if subducted
crust could penetrate the buoyancy barrier between 650
and 720 km depths (in a pyrolite mantle) it could sink
further into the lower mantle. This assumes that the
top part of the oceanic crust is representative of subducted
crust, that it is unmodified as it sinks into the mantle
(i.e. it stays unaltered and does not lose a silica-rich
melt), and that some mechanism exists for dragging it
through higher-density mantle. It also assumes that
pyrolite is a good model for the TZ and lower mantle.
Actually, Lee et al. (2004) found that the
lower mantle is 2 – 4% denser that pyrolite, which
would essentially reverse the conclusion of Hirose
et al. (1999) regarding the ability of eclogite
to sink into the lower mantle. Cammarano et al.
(2005) determined that a pyrolite lithology has higher
seismic velocities in the TZ and below, than are obtained
from seismology. This suggests a more garnetitic mineralogy
in the TZ than pyrolite and, perhaps, more FeO. The
seismic data also suggest a gradient with depth in the
TZ, with, possibly, more high-pressure olivine phases
at the bottom. The TZ also appears to vary laterally
(Ishii & Tromp, 2004).
The
lower mantle
The
controversy
There has been much controversy over
the composition of the lower mantle. Ringwood
(1975, 1994) and Kesson et al. (1994, 1998)
advocated a chemically uniform pyrolite mantle with
Mg/Si of about 1.5. This composition, however, has a
density deficit with respect to geophysical mantle models.
Others have shown that the geophysical data are consistent
with a pyroxene-rich or an iron-rich lower mantle (e.g.,
Anderson, 1977; Butler & Anderson,
1978; Stixrude et al., 1992; Stacey &
Isaak, 2000; Lee et al., 2004). In an
attempt to resolve the density discrepancy, Ringwood
(1975) suggested that enstatite might transform to MgSiO3
(perovskite) with a density 3 – 7% greater than
the isochemical mixed oxides. This transformation was
subsequently found, but the density increase was only
2% relative to the mixed oxides at zero pressure and
negligible at high pressure. The pyrolite density discrepancy
is pertinent to the question of whether eclogite will
sink into the lower mantle. Anderson (1989b)
argued that it would not, using geophysical estimates
of lower mantle density. Ringwood (1975,1994)
argued that it would, using a theoretical pyrolite density
for the lower mantle. Others have argued for a very
cold lower mantle, assuming that this could raise the
density enough for pyrolite to satisfy the geophysical
data.
Constraints
from 1D models
Cammarano et al. (2005) tested
the compatibility of a constant pyrolite composition
for the mantle, including the effects of phase changes.
The pyrolite model has seismic velocities that are too
low above 400 km, a velocity jump that is too large
at 410 km, TZ gradients that are too low, a velocity
jump at 650 km that is too small, and too strong a gradient
below the discontinuity. It appears to be difficult
to reconcile a pyrolitic mantle with global seismic
data for the TZ, given current mineral physics constraints.
Similarly, the geophysical data below 650 km are not
consistent with a chemically uniform mantle; a pyrolite
composition requires unreasonably low deep-mantle temperatures.
There is some trade-off between temperature
and composition. If a simple mixture of perovskite and
magnesiowüstite is assumed, the total iron content
of the lower mantle is found to be much greater than
the upper mantle, and Mg/(Fe + Mg) = 0.78 independent
of temperature (Stacey & Isaak, 2000).
Those authors considered this to be an implausibly high
iron content and attributed it to neglect of the presence
of Ca perovskite. There is no cosmochemical, petrological,
geochemical or geophysical prohibition against such
iron contents, except that this would preclude whole
mantle convection. They concluded that Ca-perovskite,
which they did not treat, must be an important constituent
of the lower mantle and that it is seismologically conspicuous.
Others have argued that CaSiO3- perovskite
is seismically invisible, having similar properties
to Mg-perovskite or the lower mantle, or that there
is little CaO or Al2O3 in the
lower mantle because of accretional differentiation
(Anderson, 1989a). Lee et al. (2004)
used a more realistic composition, which included pyrolitic
portions of calcium. They determined the high-pressure
mineral assemblage of an undepleted natural peridotite
– thought to be representative of the Earth’s
upper-mantle – using high-resolution X-ray diffraction.
The measured room-temperature bulk properties of this
high-pressure assemblage, together with a range of estimates
of thermal properties of the constituent minerals, appear
to be inconsistent with seismological constraints on
the density and bulk modulus of the lower mantle. Their
results suggest that the lower mantle differs in bulk
composition (e.g., is richer in iron) from
current estimates for the upper mantle.
One way of satisfying the observed
properties of the lower mantle with a pyrolite-like
composition is to invoke a higher iron content than
the preferred value for the upper mantle (Mg# = 0.90).
The density deficit of ~2-4% obtained can then be explained
by an increased abundance of iron, to an Mg# ~ 0.85.
The result is non-unique; there are tradeoffs between
temperature and composition (e.g., Si, Al and
Ca abundances) for satisfying the observed properties
of the lower mantle. Nevertheless, the intrinsic density
difference between such an iron-enriched composition
and pyrolite-like estimates of upper-mantle bulk composition
is sufficient to stabilize layered convection. An increasing
FeO content with depth in the lower mantle is also indicated.
A different lower mantle composition
results if one assumes that the Earth differentiated
during accretion, and that the lower mantle is the refractory
residue after removal of crustal and upper mantle materials,
including most of the basaltic elements (Anderson,
1989a). The depleted refractory residue is high in Si
and low in Ca and Al. A lower mantle with a Mg/Si ratio,
1.07, or 20% lower than pyrolite, and a Fe/Mg ratio
20% higher than pyrolite satisfies the density and elastic
properties of the lower mantle (Kanani Lee, personal
communication, March, 2005). An FeO-rich lower mantle
is also consistent with an enstatite chondrite protolith
for the Earth.
Tomographic
Constraints (Lateral Variations)
Ishii & Tromp (2004) determined
that density variations have a negative or nearly zero
correlation with shear- and compressional-wave seismic
velocity variations in the TZ where the root-mean-square
density amplitude is, however, high. They also showed
that the TZ velocity and velocity-density correlations
are completely different from the overlying and underlying
regions. This is inconsistent with thermal variations
or with whole-mantle convection. Subduction of cold
eclogite into the TZ, however, will lower the shear-wave
seismic velocity there (Figure 1), but will have little
effect on the density (the eclogite displaces similar
density material). An eclogite-rich TZ may also explain
the velocity jumps at 410 km and 650 km which are too
small and too large, respectively, to be entirely due
to phase changes in pyrolite (Cammarano et al.,
2005). Garnet does not undergo a phase change near 400
km so it dilutes the jumps created by phase transitions
in olivine and orthopyroxene at depths of 400 and 500
km.
Gu et al. (2001) (see also
Mantle
convection page) showed that, near 650 km depth,
there is a distinct change in seismic velocity variations,
with large variations above and small variations below.
The low shear-wave seismic velocity of eclogite and
its low melting temperature are expected to create large
tomographic variations, particularly since its distribution
is not expected to be uniform.
Slab
penetration?
What is one to make of the general
consensus in the non-seismological community that both
slabs and plumes have been imaged from the surface of
the Earth to the core-mantle boundary? One way to approach
this, for a non-specialist, is to look at a large number
of tomographic maps at different depths and a large
number of more-or-less randomly oriented cross-sections
that have not been cropped or color-saturated to make
a particular point. See, for example, Ritsema
(2005) and the supplementary
figures that accompany this paper. Many of these
cross-sections cross slabs and proposed sites of deep
mantle plumes but they usually show little continuity
below 650 km. The impression one gets from these cross-sections
is quite different from that imparted by inspection
only of the relatively small number of widely reproduced
ones that apparently show deep slab penetration.
It is useful to know how tomography
works (see also Seismology:
The hunt for plumes). In most body-wave studies
the anomaly found at a given seismic station is initially
distributed uniformly along the ray, with the expectation
that, with the addition of enough data, the anomaly
can be better localized. For a set of earthquakes in
a given region, recorded at a local array of seismic
stations, the ray bundle will be shaped like a banana,
narrow at both ends and wide in the middle where the
rays reach their deepest points. If the earthquakes
are in a cold slab and the stations on a stable continent,
then the banana will be fast – a blue banana.
If the earthquakes are on midocean ridges, or in tectonically
active continental regions, and recorded on stations
in the latter areas – where most seismometers
are – or on oceanic island stations, the banana
will be slow – a red banana. If there are few
rays that cross the banana from other directions, then
the banana will retain the color imparted to it from
the majority of the rays. The bananas show up best in
cross-sections that include the bulk of the stations
and sources. Other cross-sections will tend to show
blobs and it is not so obvious that they are the result
of smearing along ray bundles.
For example, there are many rays from
earthquakes in South American subduction zones to stations
on the Canadian Shield that sample the midmantle under
the Americas and the western Atlantic but few rays from
the Pacific or the Atlantic to help cancel out the upper
mantle (both velocity and anisotropy) effects. Likewise,
most of the data for ocean-island stations are from
steeply incident rays from distant earthquakes. Since
we know from surface-wave studies that most of the oceanic
mantle down to 600 km is low-velocity, it will be no
surprise that ocean-island stations appear to be associated
with low-velocity anomalies. Randomly placed ocean-bottom
seismometers would also be expected to yield anomalies
that resemble low-velocity, nearly vertical bananas.
However, it is islands where most of the stations are
located. Finding ways to cancel out the effects at the
ends of the bananas, and to localize the deep mantle
parts of the anomalies is an ongoing challenge to seismologists.
Using surface waves, and surface bounces such as PP
and SS helps. Methods have yet to be developed
for assessing artifacts and for judging which features
of tomographic models are believable. Forward tests
of complex synthetic models are useful, i.e. datasets
are generated for known models, which are then inverted
using current techniques.
Thermal
constraints
In a chemically stratified mantle,
with periodic injections of cold slabs and warm delaminated
continental crust, the temperature gradient will be
complex; it will not be a simple adiabat. In particular,
deep mantle temperatures will be higher than in a homogenous
convecting mantle. Mattern et al. (2005) found
that very low mantle temperatures are required for a
uniform pyrolite composition mantle. Inferred temperatures
are up to 600 K hotter for models based on cosmic abundances.
They inferred a subadiatic temperature gradient from
660 to 1300 km that correlates with a decreasing iron
content. They found no clear indication for a deeper,
chemically distinct layer or compositional boundary
as proposed by Kellogg et al. (1999) and van
der Hilst & Karason (1999). In the region from
800 to 1300 km, the mantle is significantly heterogeneous.
Between 1300 km and 2000 km depth the gradients of seismic
velocity are consistent with a homogeneous and adiabatic
mantle.
Discussion
Most continental flood basalts occur
in sutures, old orogenic belts and adjacent to thick
cratonic lithosphere, a. They form as continents either
split up or are in the final stages of convergence.
I suggest that these regions may be prone to deep crustal
removal [e.g., Kay & Kay, 1993; see also
Suzanne
Kay's home page]. The upwelling of asthensosphere
into the gap creates an initial pulse of magmatism,
as has been widely discussed. What has not been discussed
is the fate of the delaminated crustal material. What
would be evidence for delaminated material in the mantle?
First of all, it originated in the middle of a thermal
boundary layer and is not particularly cold. Second,
eclogite has higher densities but lower seismic velocities
than other upper mantle rocks, so it would show up as
a low-velocity feature in tomographic images, even while
cold. Third, eclogite is fertile, and has a lower melting
point than "normal" mantle. In summary, it
does not look like a slab seismically, and will not
act as a slab.
There is a variety of evidence supporting
the view that the outer 1000 km of the mantle is heterogeneous,
both radially and laterally. Some of this may be due
to a non-uniform distribution of eclogite and peridotite.
The idea that eclogite may be trapped in and above the
TZ is attractive. Trapped slab components warm up by
conduction from the surrounding mantle and their own
radioactivity (see Reheated
slabs pages). If temperatures reach the solidus
of eclogite, melting and buoyant ascent can initiate
and further buoyant decompression melting occurs as
the material rises. Some eclogites may equilibrate at
depths above the TZ (Figure 1). Wherever it exists,
eclogite will create fertile patches with low melting
point, and its presence will eliminate the need for
excess temperature to explain melting anomalies and
low-velocity zones. There is also no longer any need
to involve the deep mantle to explain melting anomalies.
The rationales for the plume hypothesis include the
view that the upper mantle is entirely homogeneous and
well stirred and that only high temperature can create
melting and low-velocity zones; this need not be the
case.
Delaminated lower lithosphere [Ed: Click here
for explanation of lithospheric delamination] is expected
to be refractory, chemically buoyant and only dense
while cold. The lower crust, on the other hand, becomes
intrinsically denser than the surrounding mantle and
is also fertile. Since the liquidus of eclogite occurs
at about about the same temperature as the solidus of
peridotite, I expect that mantle eclogite blobs can
become almost entirely molten as they warm up to ambient
mantle temperatures. They become buoyant when about
half the garnet is consumed, however. Deep low-velocity
zones in the mantle may be due to sinking or neutrally
buoyant eclogite rather than hot upwellings. Lower crustal
delamination (e.g., Lustrino,
2005) may explain the low-velocity zones in the
upper mantle in places such as the Ontong Java Plateau,
Yellowstone-Snake River Plain, and the Parana and the
deep low-velocity zones found atop the 410- and 650-km
discontinuities. The low-velocity features found beneath
some hotspots may be due to the re-emergence of these
fertile blobs.
|
References
& bibliography
Where
is the Eclogite?
-
Anderson,
Don L., 1967, Phase changes in the upper mantle,
Science, 157, 1165-1173.
-
Anderson,
Don L., 1979, The upper mantle TZ: Eclogite?,Geophys.
Res. Lett., 6, 433-436.
-
Anderson,
Don L., 1987, The Depths of mantle reservoirs, in
Magmatic Processes, ed. B.0. Mysen, Spec.
Publ. No. 1, Geochem. Soc.
-
Anderson,
Don L., 1989a, Theory of the Earth, Blackwell
Scientific Publications, Boston, 366 pp.
-
Anderson,
Don L., 1989b, Where on Earth is the Crust?, Physics
Today, 42, 38-46.
-
Gasparik,
T., 1997, A model for the layered upper mantle,
Phys. Earth Planet. Int., 100,
197-212.
-
Hirose, K.
F. Yingwei, Y. Ma and H.-K. Mao, 1999, The fate
of subducted basaltic crust in the Earth's lower
mantle, Nature, 397, 53-56.
-
Irifune, T.
and A.E. Ringwood, 1993, Phase transformations in
subducted oceanic crust and buoyancy relationships
at depths of 600±800 km in the mantle,
Earth Planet. Sci. Lett., 117,
101-110.
-
Kesson, S.E.,
J.D. Fitz Gerald and J.M. Shelley, 1994, Mineral
chemistry and density of subducted basaltic crust
at lower-mantle pressures, Nature, 372,
767-769.
-
-
McDonough
W. F., 1991, Partial melting of subducted oceanic
crust and isolation of its residual eclogitic lithology,
Phil. Trans. R. Soc. Lond. Series A, 335,
407-418.
-
Ringwood,
A. E., 1994, Role of the transition zone and 660
km discontinuity in mantle dynamics, Phys. Earth
Planet. Int., 86, 5-24.
-
Ringwood,
A.E., 1975, Composition and Petrology of the
Earth’s Mantle, McGraw-Hill, New York,
618 pp.
-
Rudnick R.L.,
M.G. Barth, W.F. McDonough and I. Horn, 1998, Rutiles
in eclogites: a missing Earth reservoir found? GSA
abstr. 30(7), Toronto, A-207.
Lower
Mantle
-
Anderson,
Don L., 1977, Composition of the mantle and core,
Ann. Rev. Earth Planet. Sci., 5,
179-202.
-
Anderson,
Don L., 1984, Chemical inhomogeneity of mantle above
670 km transition, Nature, 307,
114.
-
Butler, R.,
and Don L. Anderson, 1978, Equation of state fits
to the lower mantle and outer core, Phys. Earth
Planet. Int., 17, 147-162.
-
Dziewonski,
A.M. and Don L. Anderson, 1981, Preliminary reference
Earth model, Phys. Earth Planet. Int.,
25, 297– 356.
-
Helffrich,
G.R. and B.J. Wood, 2001, The Earth’s mantle,
Nature, 412, 501–507.
-
Jackson, I.,
1998, Elasticity, composition and temperature of
the Earth’s lower mantle: a reappraisal, Geophys.
J. Int., 134, 291–311.
-
Javoy, M.,
1995, The integral enstatite chondrite model of
the Earth, Geophys. Res. Lett., 22,
2219-2222.
-
Jeanloz, R.
and E. Knittle, 1989, Density and composition of
the lower mantle, Phil. Trans. Royal Soc. London,
Series A: Mathematical and Physical Sciences,
328, 377–389.
-
Kellogg, L.H.,
B.H. Hager and R.D. van der Hilst, 1999, Compositional
stratification in the deep mantle, Science,
283, 1881-1884.
-
Lee, K.K.M.,
B. O’Neill, W.R. Panero, S.-H. Shim, L.R.
Benedetti and R. Jeanloz, 2004, Equations of state
of the highpressure phases of a natural peridotite
and implications for the Earth’s lower mantle,
Earth Planet. Sci. Lett., 223,
381– 393.
-
Mattern, E.,
J. Matas, Y. Ricard and J. Bass, 2005, Lower mantle
composition and temperature from mineral physics
and thermodynamic modeling, Geophys. J. Int.,
160, 973-990.
-
McKenzie,
D.P. and F. Richter, 1981, Parameterized thermal
convection in a layered region and the thermal history
of the Earth. J. Geophys. Res., 86,
11667-11680.
-
O’Neill,
B. and R. Jeanloz, 1990, Experimental petrology
of the lower mantle: a natural peridotite taken
to 54 GPa, Geophys. Res. Lett., 17,
1477–1480.
-
O’Neill,
B. and R. Jeanloz, 1994, MgSiO3-FeSiO3-Al2O3
in the Earth’s lower mantle: perovskite and
garnet at 1200 km depth, J. Geophys. Res.,
99, 19901-19915.
-
Ringwood,
A.E., 1975, Composition and Petrology of the
Earth’s Mantle, McGraw-Hill, New York,
618 pp.
-
Rudnick, R.L.,
M. Barth, I. Horn and W.F. McDonough, 2000, Rutile-bearing
refractory eclogites: Missing link between continents
and depleted mantle, Science, 287,
278-281.
-
Stacey, F.D.,
1998, Thermoelasticity of a mineral composite and
a reconsideration of lower mantle properties, Phys.
Earth Planet. Int., 106, 219-236.
-
Stixrude,
L., R.J. Hemley, Y. Fei and H.K. Mao, 1992, Thermoelasticity
of silicate perovskite and magnesiowustite and stratification
of the Earth’s mantle, Science, 257,
1099-1101.
-
Stixrude,
L. and M.S.T. Bukowinski, 1992, Stability of (Mg,Fe)SiO3
perovskite and the structure of the lowermost mantle,
Geophys. Res. Lett., 19,
1057-1060.
-
Stacey, F.
and D.G. Isaak, 2000, Extrapolation of lower mantle
properties to zero pressure: Constraints on composition
and temperature equation of state of a natural upper-mantle
rock at conditions of the Earth’s lower mantle,
American Mineralogist, 85,
345-353.
-
van der Hilst,
R.D. and H. Karason, 1999, Compositional heterogeneity
in the bottom 1000 kilometers of Earth’s mantle:
toward a hybrid convection model, Science,
283, 885-1888.
Transition
Zone
-
Cammarano,
F., A. Deuss, S. Goes and D. Giardini, 2005, One-dimensional
physical reference models for the upper mantle and
transition zone: Combining seismic and mineral physics
constraints, J. Geophys. Res., 110,
B1, B01306, 10.1029/2004JB003272.
-
Duffy, T.S.,
and Don L. Anderson, 1989, Seismic velocities in
mantle minerals and the mineralogy of the upper
mantle, J. Geophys. Res., 94,
1895-1912.
-
Fukao, Y.,
S. Widiyantoro and M. Obayashi, 2001, Stagnant slabs
in the upper and lower mantle transition region,
Rev. Geophys., 39, 291-323.
-
Gu, Y., A.M.
Dziewonski, S. Weijia and G. Ekstrom, 2001, Models
of the mantle shear velocity and discontinuities
in the pattern of lateral heterogeneities, J.
Geophys. Res., 106, 11,169-11,199.
-
Ishii, M.
and J. Tromp, 2004, Constraining large-scale mantle
heterogeneity using mantle and inner-core sensitive
normal modes, Phys. Earth Planet. Int.,
146, 113–124.
-
Kesson, S.E.,
J.D. Fitz Gerald and J.M. Shelley, 1998, Mineralogy
and dynamics of a pyrolite lower mantle, Nature,
393, 252-255.
-
Kesson, S.E.,
J.D. Fitz Gerald and J.M. Shelley, 1994, Mineral
chemistry and density of subducted basaltic crust
at lower-mantle pressures, Nature, 372,
767-769.
Mixing
and Stirring
-
Christensen,
U.R., 1989, Models of mantle convection - one or
several layers, Phil. Trans. R. Soc. London
Series A, 328, 417-424.
-
Meibom, A.
and Don L. Anderson, 2003, The Statistical Upper
Mantle Assemblage, Earth Planet. Sci. Lett.,
217, 123-139.
-
Olsen, P.L.,
D.A. Yuen and D.S. Balsiger, 1984, Mixing of passive
heterogeneities by mantle convection, J. Geophys.
Res., 89, 425-36.
-
McKenzie,
D.P. and F. Richter, 1981, Parameterized thermal
convection in a layered region and the thermal history
of the Earth, J. Geophys. Res., 86,
11667-11680.
-
van Keken,
P.E., E.H. Hauri and C.J. Ballentine, 2002, Mantle
mixing: the generation, preservation, and destruction
of chemical heterogeneity, Ann. Rev. Earth Planet.
Sci., 30, 1-33.
Delamination
-
Kay, R.W. and S.M.
Kay, 1993, Delamination and delamination magmatism,
Tectonophysics, 219, 177-189.
-
Kay, R.W. and S.M.
Kay, 1992, Delamination magmatism and shallow, hot
subduction zone magmatism: Implications for crust-mantle
evolution, 29th International Geologic Congress,
Japan.
-
Lustrino, M., E.
Mascia and B. Lustrino, 2004. EMI, EMII, EMIIE,
EMIII, HIMU, DMM, et al. What do they really mean?
32nd International Geological Congress, Florence,
Italy, 2004, abs. Vol., pt. 1, abs. 170-23.
-
Lustrino,
M. and M. Wilson, 2005. The circum-Mediterranean
anorogenic Cenozoic igneous province, Earth
Sci. Rev. (submitted).
-
|
last updated 23rd
June, 2005 |
|
|