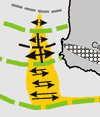 |
Shallow
magma sources during continental rifting and
breakup of the South Atlantic |
Dieter
Franke, Karl Hinz, Stefan
Ladage, Soenke
Neben*, Michael
Schnabel, Bernd
Schreckenberger
Federal Institute
for Geosciences and Natural Resources, Hannover, Germany
(BGR)
*Our research partner, kind and valued
friend Soenke Neben passed away suddenly and unforeseen
in his forty-seventh year on November 13th, 2009
Dieter.Franke@bgr.de ; geohinzhannover@aol.com ; Stefan.Ladage@bgr.de ; Michael.Schnabel@bgr.de ; Bernd.Schreckenberger@bgr.de
Click here to
visit our on-line database
This webpage is a synopsis of the papers:
- Franke, D., Neben, S., Ladage, S., Schreckenberger,
B., Hinz, K., 2007. Margin
segmentation and volcano-tectonic architecture along
the volcanic margin off Argentina/Uruguay, South
Atlantic, Marine Geology, 244/1-4,
pp. 46-67. doi:10.1016/j.margeo.2007.06.009
- Franke, D., S. Ladage, M. Schnabel, B. Schreckenberger,
C. Reichert, K. Hinz, M. Paterlini, J. de Abelleyra,
and M. Siciliano, 2010. Birth
of a volcanic margin off Argentina, South Atlantic, Geochem.
Geophys. Geosyst., 11, Q0AB04,
doi:10.1029/2009GC002715.
Introduction
The precursor of the plate tectonic
theory, the continental drift hypothesis [Wegener,
1912], was initially pushed by the astonishing geometrical
fit of shelf edges of the South Atlantic. Consequentially,
the southern South Atlantic allowed a first reassembly
accomplished by sequentially fitting pairs of continents
after determining their best fitting poles of rotation
[Bullard et al., 1965]. In particular the
region between the Falkland-Agulhas Fracture Zone and
the Rio Grande Rise/Walvis Ridge is undisturbed by
major regional jumps of the spreading axis and there
are no complex oceanic features, such as volcanic ridges
or volcanic plateaus (Figure 1). The opening of the
South Atlantic occurred diachronously, rejuvenating
from south to north [e.g., Rabinowitz,
1976; Rabinowitz & Labrecque, 1979; Austin & Uchupi,
1982; Sibuet et al., 1984; Uchupi,
1989] and may be described as a progressive northward
unzipping of rift zones [e.g., Nürnberg & Müller,
1991; Jackson et al., 2000].
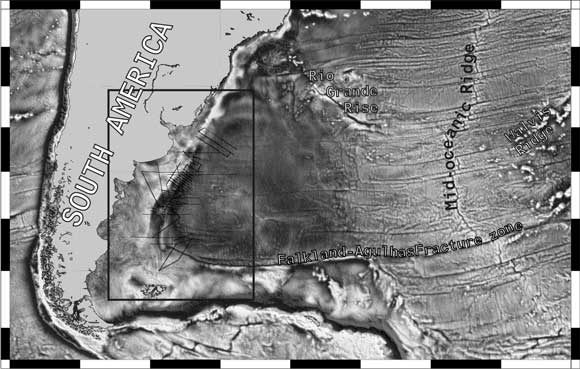
Figure 1: Overview map showing
the western South Atlantic and the study area between
the Falkland-Agulhas Fracture Zone and the Rio Grande
Rise/Walvis Ridge.
The South Atlantic margins are of
the rifted volcanic margin type [Hinz, 1981],
as are the majority of passive margins worldwide. The
Early Cretaceous South Atlantic continental break-up
and initial sea-floor spreading were accompanied by
extensive transient volcanism and magmatism recorded
as inferred sill intrusions, flood basalt sequences,
voluminous volcanic wedges, and magmatic underplating.
In seismic reflection data the voluminous extrusives
are manifested by huge wedges of seaward dipping reflectors
(SDRs) on both sides of the southern South Atlantic
[Gladczenko et al., 1997; Hinz et al.,
1999; Franke
et al.,
2007; Franke
et al., 2010].
Here we report a detailed investigation
of the Argentina and Uruguay outer margin segments
based on a set of about 25.000 line-kilometres of 2D
multichannel seismic data that were acquired by BGR
during the past 20 years (Figure 2). Synthesis of these
data show that the SDR formations vary extensively,
yet systematically in architecture, extent and thickness
along the strike of the margin. We conclude that the
emplacement of the now deeply buried, 60-120 km wide
SDRs occurred episodically. The overall northward propagation
of the South Atlantic rift took place in huge (400
km scale), but distinct along-margin segments that
are bounded by transfer zones. Each segment reveals
internally the same trend of northward decreasing volume
of emplaced extrusives.
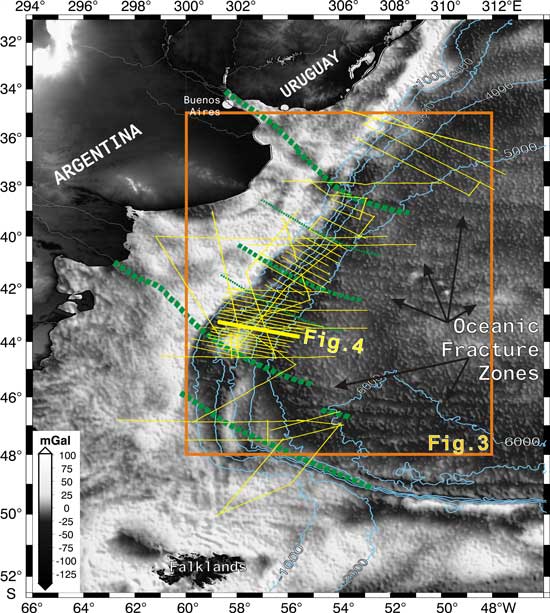
Figure 2: Study area in the western
South Atlantic and location of multichannel seismic
reflection lines (yellow lines) of BGR cruise 1987,
R/V SONNE cruise SO-85 1993, BGR cruise 1998 and
BGR cruise 2004. The location of the example seismic
lines shown in Figure 4 is indicated as thick yellow
solid line. Satellite-derived gravity field (Sandwell & Smith,
1997) is shown for the offshore area. Proposed transfer
zones as interpreted in Figure 3 are indicated as
dashed green lines. Oceanic fracture zones in the
deep Argentine Basin may correlate with the suggested
transfer zones.
Margin
segmentation and style of the emplaced volcanics
Four major transfer zones on the volcanic
Argentine/Uruguayan margin, South Atlantic, have been
identified [Franke
et al.,
2007; Figure 3]. It is suggested that the margin
can be divided into at least four compartments (Segments
I to IV) bounded by the Falkland Fracture Zone/Falkland
transfer, the Colorado transfer, the Ventana transfer
and the Salado transfer. Criteria for mapping and extending
transfer zones across the margin were:
- a major lateral offset in the distribution of
the SDR wedges is observed,
- the basement shows a steeper than average slope
with, in most cases, steep, deep penetrating faults
offsetting the base of the post-rift sediments,
and
- either the architecture and style of the SDR
wedges changes dramatically, or indications of
SDRs are weak or absent.
Figure 3: Structural map showing
the distribution of extensive volcanics manifested
by thick wedges of seaward dipping reflector sequences
(SDRs), additional volcanic/magmatic features and
oceanic basement depressions. Transfer zones and
margin segmentation as interpreted from variations
in the margins volcano-tectonic suite, as well as
post-rift sediment distribution, potential field
data and earlier studies.
According to our interpretation the
transfer zones probably represent old zones of weakness
controlling the onset of the Upper Cretaceous seafloor
spreading and may be linked and extending to recent
oceanic fracture zones (Figure 2).
Our data confirm other studies that
found considerable variations in the seismic character
of SDRs [Franke
et al., 2007]. Distinct unconformities with
a low-frequency seismic pattern, separating the SDR
wedges, are concentrated in margin segments II and
III (Figures 3 & 4). The individual flows are spatially
separated in the south while the main SDR wedge 1 becomes
increasingly buried beneath main SDR wedge 2 in the
northern part of these segments. In the northern part
of margin segment II one broad wedge is present with
an arcuate, high frequency internal pattern. This is
replaced by three spatially separated wedges of SDRs
where the main wedge is offset in a sinistral sense
by 20 to 30 km at the Ventana transfer. In margin segment
IV we observe again spatially separated individual
flows bounded by strong unconformities. These variations
are the expression of an apparently general trend in
the breadth and thickness of the SDR sequences: The
largest volumes of volcanics are systematically emplaced
along the southern edges of the margin segments, just
north of the transfer zones. The breadth and thickness
of the SDRs decrease towards the north up to the next
transfer zone. Another wide and thick wedge was emplaced
at the southern edge of the segment adjacent to the
north.
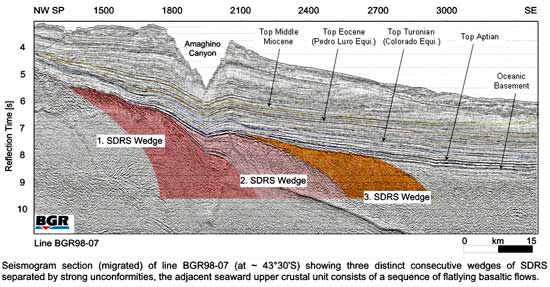
Figure 4: Profile BGR98-07, situated
in margin segment II. Multiple SDRs wedges are separated
by strong unconformities. The top of the oceanic
crust is well defined by a flat, low-frequency reflector
band. Click here or
on figure for enlargement.
Distribution of
the volcanics along one margin segment
In the following we report on a detailed
investigation of the volcanic/magmatic edifices across
the margin and their variations along one margin segment
[Franke
et al., 2010].
We concentrate on a segment between the Colorado and
the Ventana transfer zones, between 41°S and 44°S.
Within the margin segment SDRs are
continuously present along strike. However, as Table
1 shows, the SDRs vary significantly in extent, thickness
and volume. Although large uncertainties exist in the
volume calculation, a considerable northward decrease
of the volume of eruptives is obvious.
Table 1: Extent of the Inner SDRs
in margin segment II. The position is given for the
intersection of the strike lines with the margin-parallel
line BGR98-26. Line BGR98-43 did not reach the seaward
end of the SDRs.
Line
No
Survey
BGR98 |
LatitudeCenter
SDR [°S] |
Extent
of SDRs across the margin [km] |
Max.
thickness twt [s] |
Max.
thickness [km] |
Cross-sectional
area, km2 |
|
aligned
along line 26 |
|
|
(at
V = 5.8 km/s) |
(assuming
right-angle triangle shape) |
North |
41 |
41.74 |
|
|
33 |
2.4 |
7.0 |
115 |
42 |
41.93 |
|
|
40 |
2.0 |
5.8 |
116 |
43 |
42.21 |
|
|
?34+x |
2.1 |
6.1 |
?104+x |
14 |
42.39 |
|
|
53 |
2.3 |
6.7 |
177 |
15 |
42.55 |
|
|
47 |
2.4 |
7.0 |
164 |
|
|
SDR
wedge 1 |
+SDR
wedge 2 |
+SDR
wedge 3 |
|
|
|
16 |
42.71 |
15 |
32 |
44 |
2.4 |
7.0 |
153 |
17 |
42.87 |
19 |
35 |
58 |
2.4 |
7.0 |
202 |
05 |
43.03 |
21 |
37 |
58 |
2.6 |
7.5 |
219 |
18 |
43.15 |
26 |
37 |
46 |
2.6 |
7.5 |
173 |
19 |
43.25 |
29 |
41 |
47 |
2.2 |
6.4 |
150 |
06 |
43.36 |
34 |
48 |
56 |
2.1 |
6.1 |
171 |
07 |
43.53 |
38 |
57 |
72 |
2.0 |
5.8 |
209 |
08 |
43.70 |
57 |
79 |
98 |
2.0 |
5.8 |
284 |
20 |
43.80 |
57 |
79 |
100 |
2.0 |
5.8 |
290 |
09 |
43.88 |
56 |
78 |
93 |
1.8 |
5.2 |
243 |
21 |
43.99 |
57 |
80 |
88 |
1.8 |
5.2 |
230 |
South |
Multiple Inner SDR wedges are distinct
in the southern part of the segment. In the south the
Inner SDR domain is up to 100 km wide in the east–west
direction. The vertical thickness increases from 1.8
s (TWT) to a maximum value of 2.6 s (TWT) where multiple
wedges show maximum overlap, at the northern limit
of the multiple SDR occurrence. Further north, one
broad, 30 to 53 km wide SDR wedge is present with an
arcuate, high-frequency internal pattern. Here, the
SDR wedge can be traced down almost to Moho depth where
it is not masked by multiples and appears to terminate
against horizontal reflections, about 1 s (TWT) above
the Moho.
The fact that the multiple Inner SDR
wedges off Argentina were emplaced rather side by side
and overlap to a certain degree provides strong evidence
for multiple phases of volcanism. This is similar to
the Norwegian volcanic margin, where Eldholm et
al. [1986] suggested that overlapping successions
of SDRs were emplaced by consecutive phases of volcanism.
Other indicators for episodic volcanic activity off
Argentina are certain characteristics of the magnetic
anomalies. The magnetic signature in the area of the
SDRs changes both along and across the margin. North
of the Colorado transfer zone, in the area of the multiple
SDR wedges, several magnetic anomalies and polarity
reversals are observed, indicating that the individual
flows were emplaced with different polarities.
According to our interpretation, the
SDRs furthest west and now furthest landward were emplaced
first. Eventually one or several intrusions reached
the surface and resulted in this volcanic flow unit.
During a period of magmatic stagnation erosion and
weathering affected the top of this flow resulting
in an unconformity. Continuing extension resulted in
thinning of the crust but a stable magma chamber did
not yet develop. Before the next volcanic pulse was
initiated, the injection center migrated east and the
second wedge was emplaced next to the first. As before,
this flow unit was exposed and eroded before the next
flow was emplaced from an injection center located
even farther east. In the south, this second flow partly
covers the first wedge, but the main part of this wedge
is located seaward of the first SDR flows. Towards
the north, the main SDR wedge 1 decreases in width
and becomes increasingly buried beneath main SDR wedge
2. SDR wedge 2, in contrast, shows a continuous or
slightly increasing width and wedge 3 is irregularly
distributed.
Evolution of the
volcanic rifted margin
At the northern edge of the magma-poor
margin segment, at ~44°S, an irregular crustal
reflection pattern is present, which may be related
to an evolving feeder dyke system. There may be an
interbedding of volcanoclastics, tuff and ashes between
this reflection and the basement reflection, but no
distinct SDRs developed. Across the Colorado transfer
zone, a transition from magma-poor to magma-rich rifting
takes place within about 10 kilometers. Remarkably,
the widest SDR wedges are found close to this transition,
at the northern edge of the transfer zone. This abrupt
change in emplaced magmatic volume leads us to consider
alternative scenarios to the hypothesis that gradual
along-margin variations in the thermal regime of the
lithosphere and sublithospheric mantle (the traditional
plume-driven model) are solely responsible for the
transition from magma-poor to magma-rich volcanic margins.
Gradual changes of mantle properties and dynamics would
be expected to generate a smooth transition from magma-starved
to volcanic rifting over at least a hundred or a few
hundreds of kilometers [Franke
et al., 2010].
Additional findings that do not support
this hypothesis (or its application to the Argentine
margin), include the episodic emplacement of the SDRs
and the proposed seaward migrating injection center
for the SDRs during rifting. If the region were underlain
by a stable, long-lasting thermal anomaly driving the
extension, why would multiple phases of volcanism alternate
with periods of magmatic stagnation? Such periods of
stagnation are the best explanation for the presence
of unconformities on top of the SDRs and the varying
magnetic signals of the SDR wedges. Finally, northward-decreasing
volumes and production rates of melts as manifested
by the SDR units (Table 1) are difficult to reconcile
with the idea that the Tristan da Cunha plume caused
the volcanic/magmatic edifices off Argentina. This
plume hypothesis would predict a decrease of volcanism
and magmatism with increasing distance from the plume
head.
Where was the
melt generated?
The production of the large volumes
of basaltic magma as manifested by the SDR sequences
in volcanic rifted margins is certainly spatially and
temporally related to continental breakup. However,
the mechanism responsible for the emplacement of the
basaltic flows is quite controversial [e.g., Menzies
et al., 2002]. Among other factors, melts are
produced by variations in pressure and temperature.
Changes in these parameters can be achieved by either
lithospheric thinning or plumes or, more generally
expressed, thermal anomalies.
The Paraná-Etendeka continental
flood basalt provinces in Brazil and Namibia, respectively,
were emplaced mainly between 129 and 133 Ma [e.g., Renne
et al., 1992; Stewart et al., 1996; Peate, 1997; Menzies
et al., 2002]. Newer studies from the western
margin of the Paraná province reveal that melt
generation occurred in two major phases; at 145 Ma
and 127.5 Ma [Gibson et al., 2006]. These
authors conclude that the Paraná-Etendeka large
igneous province was associated with the impact of
the Tristan plume, it was long-lived, and it immediately
predated continental break-up.
Although these findings may be consistent
with anomalous mantle temperature influencing the evolution
of the volcanic margin, the magmatic architecture of
the margin and the structures identified in our data
can hardly be explained by a simple plume model originating
from the deep mantle:
-
Why should the rift start in
the south at about 48°S when the plume was
centered at about 30°S?
-
If the South Atlantic opened
like a zipper from south to north, how does this
fit with the plume model?
-
Most striking is the question
of the spatial distribution of melts manifested
by the SDR sequences. Within the plume hypothesis
a continuous decrease (or, at least, a continuous
amount) of volcanism and magmatism with increasing
distance from the plume is expected.
The seismic data demonstrate that
the offshore SDRs, which are in excess of 10 kilometres
thick, are much thicker than the average flood basalt
thickness in the onshore Paraná province, which
is only 0.7 km [Franke
et al., 2007]. More important, however, is
the observation that the rate of volcanic rock production
decreases from south to north within the individual
volcanic margin segments II and III, bounded by the
Colorado, Ventana and Salado transfer zones (Figures
2 & 5). The major part of the volcanic extrusives
more or less terminates to the south at the Colorado
transfer. Some 150 km south of this location there
is another SDR wedge located beneath the slope. From
these findings we suggest a link between margin segmentation
and volume, architecture and breadth of the volcanics
for the western South Atlantic margin. The (minimum
of) four transfer zones offset the Lower Cretaceous
rift and are associated with changes in distribution
and volume of emplaced volcanic material. They mark
changes in structural pattern and margin subsidence.
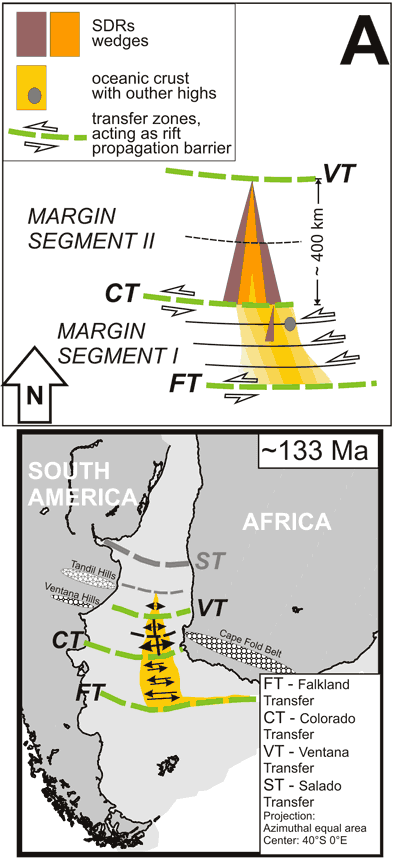
Figure 5A: Sketch illustrating
the evolution of the southern South Atlantic rift
and plate reconstructions for 133 Ma (modified from
Jokat et al., 2003 and Macdonald et al., 2003). Time
scale according to Gradstein et al. (2004). Margin
segment I between the Falkland transfer (FT) and
the Colorado transfer (CT) is dominated by strike-slip
movements, which probably prevent the generation
of large volumes of melt. North of the Colorado
transfer (CT) a longer section (margin segment II)
opens with initially a narrow SDR wedge. At the future
transfer zone about 400 km to the north (Ventana
Transfer, VT), rifting is interrupted resulting in
heat accumulation in the upper mantle. This enhances
convection in the asthenosphere and the subsequent
emplacement of multiple SDR wedges. The breadth of
the SDR wedges decreases northwards.
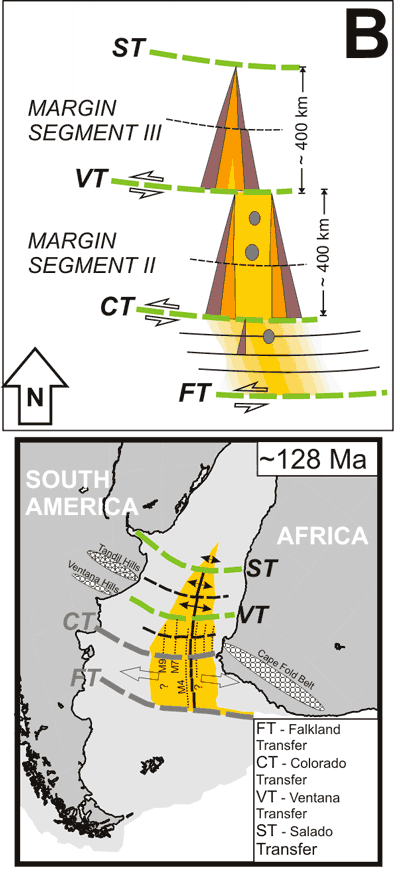
Figure 5B: Sketch illustrating
the evolution of the southern South Atlantic rift
and plate reconstructions for 128 Ma (modified from
Jokat et al., 2003 and Macdonald et al., 2003). Time
scale according to Gradstein et al. (2004). When
the next segment opened (margin segment III) north
of the Ventana transfer (VT) initially a narrow SDR
wedge was emplaced with an offset in a sinistral
sense. Another major transfer (ST - Salado transfer),
again about 400 km to the north, interrupts the rifting
process. The same heat accumulation and enhanced
mantle convection is proposed as for margin segment
II, resulting in the emplacement of the multiple
SDRs wedges in this margin segment. At this stage
we suggest an overprint of the southern segment resulting
in the formation of volcanic outer highs and SDR sequences.
As pointed out by Meyer et al. [2007]
there are unexplained aspects in the mantle plume concept
and alternative models need to be developed. We suggest
that the mode of opening of the South Atlantic substantially
influenced the varying emplacement of the volcanic
extrusives. Breakup by a successive northward unzipping
of rift zones [e.g., Nürnberg and
Müller, 1991; Jackson et al., 2000]
with a triangle-shaped opening of the ~ 400-km-long
margin segment as suggested by Franke
et al. [2007] is
expected to result in differential stretching along
the strike of the margin. This scissor-like opening
explains the fact that the injection center of the
multiple SDR wedges migrated contemporaneously with
ongoing extension in a seaward direction in the south,
while it was more fixed in the northern part of this
segment. As a consequence, in the southern part of
a margin segment the Inner SDRs were emplaced rather
side by side, whereas in their northern part they were
stacked on top of on another. Likewise the transitional
zone between the Inner and Outer SDRs narrows in a
northern direction. In this scenario phases of volcanism
correspond to high extension rates while phases of
stagnation reflect small extension rates or temporary
stagnation in the rifting process.
Lithosphere rupturing in the form
of fast propagating rift zones has been inferred as
the mechanism for transient excess melting by decompression
for the diachronous emplacement of the SDRs along the
Argentina margin [Hinz et al., 1999]. The
new data suggest that the observed transfer zones are
important features for the emplacement of the SDRs.
Interruption of the rifting process
(transfer zone) will lead to heat accumulation beneath
the thinned and stretched crust in the next segment.
This may result in enhanced mantle convection [e.g., Mutter
et al., 1988; Saunders et al., 1997],
eventually leading to a mature rifting stage with the
emplacement of huge amounts of extrusives. When the
next segment was disrupted far field stresses may have
affected the adjacent segment to the south resulting
in the emplacement of the flat lying flows and the
outer high, outer SDRs wedges (Figure 5b). The mantle
temperature may or may not have been elevated above
that of normal asthenosphere before breakup but local
melt generation by adiabatic decompression in our view
better explains the distinct variations in the architecture
of the volcanic margin. [Ed: See also Rifting
decompression melting page].
Conclusions
-
The architecture, style and
extent of the seaward dipping reflector sequences
(SDRs) vary extensively and systematically. A general
trend is that the largest volumes are emplaced
close to the mapped transfer zones and the breadth
of the SDR wedges decreases northward within the
individual margin segments.
-
Plume-driven models are the
traditional explanation for the formation of volcanic
rifted margins. However, the following observations
along the studied margin segment are difficult
to explain by this model:
- The transition from magma-poor to magma-rich
rifting takes place within only about 10 kilometers.
- The SDRs were emplaced episodically and the
injection center for the multiple Inner SDRs
likely migrated seaward during rifting.
- The volumes of melts as manifested by the
Inner SDR units decrease towards the Tristan
da Cunha plume.
-
A scissor-like opening of the
margin segment under study explains both the migrating
injection center of the multiple Inner SDR wedges
in the south and the stacked SDRs in the north.
We speculate that the varying amount of melts that
were emplaced along the strike of the margin segment
is related to the mode of opening of the South
Atlantic. Presuming that the transfer zones formed
prominent lithospheric discontinuities at the onset
of rifting, these are expected to have strongly
influenced the generation of melts. An axial-symmetrical
small-scale mantle convection system may have developed
all along the rifted margin segment [ van Wijk
et al.,
2004] with the transfer zones having
acted as rift propagation barriers. In this model
decreasing extension rates toward the north along
the margin segment under study result in decreasing
volumes of melts. We do not exclude elevated mantle
temperatures during rifting. However, we propose
that a vast amount of the volcanic/magmatic structures
found at this particular margin can be sufficiently
explained if we consider that passive rifting processes
controlled, at least partly, the production rates
of melts.
References
-
Austin, J.A., Uchupi, E., 1982.
Continental-Oceanic crustal transition of southwest
Africa. AAPG Bulletin 66,
1328-1347.
-
Bullard, E., Everett, J.E. and
Smith, A.G., 1965. The fit of continents around
the Atlantic. Phil. Trans. Roy. Soc. London 258A,
41-51.
-
Eldholm, O., et al., 1986, Formation
of the Norwegian Sea, Nature, 319,
360-361.
-
Franke, D., Neben, S., Schreckenberger,
B., Schulze, A. Stiller, M. & Kravczyk, C.,
2006. Crustal structure across the Colorado Basin,
offshore Argentina. Geophysical Journal International 165,
850-864. doi: 10.1111/j.1365-246X.2006.02907.x.
-
Franke,
D., Neben, S., Ladage, S., Schreckenberger, B.,
Hinz, K., 2007. Margin segmentation and volcano-tectonic
architecture along the volcanic margin off Argentina/Uruguay, South
Atlantic, Marine Geology 244/1-4, 46-67.
doi:10.1016/j.margeo.2007.06.009
-
Franke,
D., S. Ladage, M. Schnabel, B. Schreckenberger,
C. Reichert, K. Hinz, M. Paterlini, J. de Abelleyra,
and M. Siciliano, 2010. Birth of a volcanic margin
off Argentina, South Atlantic, Geochem. Geophys.
Geosyst., 11, Q0AB04, doi:10.1029/2009GC002715.
-
Gibson, S.A., Thompson, R.N.,
Day J.A., 2006. Timescales and mechanisms of plume–lithosphere
interactions: 40Ar/39Ar geochronology and geochemistry
of alkaline igneous rocks from the Paraná–Etendeka
large igneous province. Earth and Planetary
Science Letters 251, 1-17.
-
Gladczenko, T.P., Hinz, K.,
Eldholm, O., Meyer, H., Neben, S., Skogseid, J.,
1997. South Atlantic volcanic margins. J. Geol.
Soc. London 154, 465–470.
-
Gradstein, F.M., Ogg, J.G.,
Smith, A.G., et al., 2004. A Geologic Time
scale 2004. Cambridge University Press, Cambridge,
pp. 589.
-
Hinz, K., 1981. A hypothesis
on terrestrial catastrophes: wedges of very thick
oceanward dipping layers beneath passive continental
margins—their origin and paleoenvironmental
significance. Geologisches Jahrbuch, Reihe
E, Geophysik 22, 3–28.
-
Hinz, K., Neben, S., Schreckenberger,
B., Roeser, H.A., Block, M., Gonzalvez de Souza,
K., Meyer, H., 1999. The Argentine continental
Margin north of 48°S: Sedimentary successions,
volcanic activity during breakup. Mar. Petrol.
Geol. 16, 1-25.
-
Jackson, M.P.A., Cramez,
C., Fonck, J.-M., 2000. Role of subaerial volcanic
rocks and mantle plumes in creation of South Atlantic
margins: implications for salt tectonics and source
rocks. Marine and Petroleum Geology 17 (4),
477-498.
-
Jokat, W., Boebel, T., König,
M., Meyer, U., 2003. Timing and geometry of early
Gondwana breakup. J. Geophys. Res. 108,
doi:10.1029/2002JB001802, 1-19.
-
Macdonald, D., Gomez-Pereza,
I., Franzese, J., Spalletti,, L., Lawver, L., Gahagan,
L., Dalziel, I., Thomas, C., Trewind, N., Hole,
M., Paton, D., 2003. Mesozoic break-up of SW Gondwana:
implications for regional hydrocarbon potential
of the southern South Atlantic. Marine and
Petroleum Geology 20, 287–308.
-
Menzies, M.A., Klemperer, S.L.,
Ebinger, C.J., Baker, J. 2002. Characteristics
of volcanic rifted margins, In: Menzies, M.A.,
Klemperer, S.L., Ebinger, C.J., Baker, J. (Eds.), Volcanic
Rifted Margins. Boulder, Colorado, Geological
Society of America Special Paper 362, 1-14.
-
Meyer, R. van Wijk, J., Gernigon
L., 2007, The North Atlantic Igneous Province:
A review of models for its formation, in Plates,
Plumes, and Planetary Processes, edited by
G. R. Foulger and D. M. Jurdy, pp. 525 – 552,
Geological Society of America Special Paper, 430.
-
Mutter, J.C., Buck, W.R., Zehnder,
C.M., 1988. Convective partial melting – A
model fort he formation of thick basaltic sequences
during the initiation of spreading. Journal
of Geophysical Research 93,
1031-1048.
-
Nürnberg, D., Müller,
R.D., 1991. The tectonic evolution of the South
Atlantic from Late Jurassic to present. Tectonophysics 191,
27-53.
-
Peate, D.W., 1997. The Paraná–Etendeka
Province. In: Mahoney, J. J., Coffin, M. F. (Eds.) Continental,
Oceanic, and Planetary Flood Volcanism, Geophysical
Monograph 100, 217–245.
-
Rabinowitz, P.D., 1976. Geophysical
study of the continental margin of southern Africa. Geological
Society of America Bulletin 87,
1643-1653.
-
Rabinowitz, P.D., Labrecque,
J.L., 1979. The Mesozoic South Atlantic Ocean and
evolution of its continental margins. J. Geophys.
Res. 84, 5973-6002.
-
Renne, P., Ernesto, M., Pacca,
I., Coe, R., Glen, J., Prevot, M., Perrin, M.,
1992. The age of the Parana flood volcanism, rifting
of Gondwanaland, and the Jurassic-Cretaceous boundary. Science 258,
975-979.
-
Sandwell, D. T., Smith, W. H.
F., 1997. Marine Gravity from Geosat and ERS-1
Altimetry, J. Geophys. Res. 102,
10039-10054.
-
Saunders, A.D., Fitton, J.G.,
Kerr, A.C., Norry, M.J., Kent, R.W., 1997. The
North Atlantic igneous province. In: Mahoney, J.,
Coffin, M.F. (Eds.), Large igneous provinces:
Continental oceanic and planetary flood volcanism.
American Geophysical Union Geophysical Monograph
100, 45–94.
-
Sibuet, J.-C., Hay, W.W., Prunier,
A., Montadert, L., Hinz, K., Fritsch, J., 1984.
Early evolution of the South Atlantic Ocean: Role
of the rifting episode. In: Hay, W.W., Sibuet,
J.-C., AL E. (Eds.), Initial Reports of the
Deep Sea Drilling Project 75,
469-481.
-
Stewart, K., Turner, S., Kelley,
S., Hawkesworth, C., Kirstein, L., Mantowani, M.,
1996. 3-D 40Ar/39Ar geochronology in the Parana
flood basalt province. Earth and Planetary
Science Letters 143, 95-110.
-
Uchupi, E., 1989. The tectonic
style of the Atlantic Mesozoic rift system: J.
African Earth Sciences 8,
no. 2/3/4, 143-164.
-
-
Wegener, A., 1912. Die Entstehung
der Kontinente. Geologische Rundschau, 3,
276-292 (in German).
last updated 19th
March, 2010 |