 |
post-perovskite |
The
D" Region: The Putative Source of Large Mantle
Plumes
Recent developments |
|
A
brief discussion of the relevance of the D" Region
to the mantle plume hypothesis is given with a summary
of recent seismological constraints and speculations
on the lowermost mantle. |
If large, persistent
thermal plumes exist in Earth’s mantle, they most
likely rise from a thermal boundary layer in the interior
(e.g., What is a plume?
page ). The steep, super-adiabatic thermal gradient
within a thermal boundary layer favors the development
of boundary layer instabilities, with upwelling plumes
of hot boundary layer material winning out over conduction
as the more efficient mechanism to transport heat upward
once the boundary layer thickens to a critical level.
There is, as yet, no compelling direct evidence for
the existence of any mid-mantle thermal boundary layer,
although this possibility has not been ruled out. On
the other hand, it is generally agreed that a thermal
boundary layer does exists in the lowermost mantle,
the so-called D" Region, due to the requirement
that heat must flux out of the core in order to sustain
the geodynamo (Lay et al., 2004). It is plausible
that thermal instabilities develop in this boundary
layer. Thus, the source of any large mantle plumes is
generally assumed to be the D" Region (Lay,
2005).
Thermal plumes will drain
hot material from the boundary layer as long as they
persist, and in a homogeneous medium with temperature
dependent viscosity, the most mobile material will be
the lowest viscosity material at the base of the hot
boundary layer. Thus, one might hope to find a geochemical
signature of mantle material that has been in direct
contact with Earth’s core present in the erupted
lavas of a hotspot volcano fed by a mantle plume (see
Osmium-Tungsten page). The plume
material should also bear upward the thermal contrast
of the boundary layer itself. Estimates of the D"
temperature contrast that assume whole mantle convection
range up to as high as 1500°C (see Lay et al.,
2004), much greater than the excess temperatures inferred
for many hot spot melts. These considerations should
motivate interest in D" structure for all parties
exploring the mantle plume hypothesis.
Seismological studies
reveal substantial complexity in the seismic wave velocity
structure of D", and several recent reviews highlight
general attributes of the region (e.g., Lay
& Garnero, 2004; Lay et al., 2004).
Two key attributes of the lowermost mantle are considered
here. First is the fact that global seismic tomography
has resolved the presence of large-scale heterogeneity
in D", dominated by large regions with shear velocity
lower than average under the central Pacific and under
southern Africa, the southern Atlantic and southern
Indian Ocean. Figure 1 is a map showing the shear velocity
heterogeneity in the lowermost mantle from a representative
tomography model (Grand, 2002).
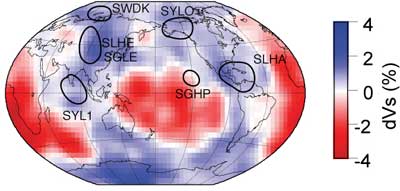 |
Figure
1. Laterally varying S-wave velocities at ~2700
km depth in the mantle from the tomography model
of Grand (2002). Blue regions: higher than average
velocity, red regions: lower than average velocity.
Average is defined by the PREM model (Figure 2).
Labeled subregions: areas where an S-wave velocity
discontinuity has been observed a few hundred
kilometers above the core-mantle boundary (CMB).
This usually corresponds to regions where seismic
velocity is higher than average, which may be
regions where temperature is lower than average,
with a post-perovskite transition at shallower
depths than in low-velocity/hotter regions. |
While there are some differences in the details
between tomography models, all are consistent
in general in terms of the large-scale pattern,
often described as a “circum-Pacific ring
of high velocities and two large superplumes”.
The existence of large-scale heterogeneities
in the thermal boundary layer in D" was
not anticipated; a hot, relatively low-viscosity
boundary layer might be expected to have a “white”
heterogeneity spectrum rather than the observed
“red” spectrum. The second key seismological
attribute is that there are many observations
of rapid increases (downward) in seismic velocities
200-300 km above the core-mantle boundary (CMB),
with somewhat greater increases being found
for shear velocity (Figure 2). Most observations
of this so-called “D" discontinuity”
are in regions where the shear velocity is higher
than average in global tomographic models (Figure
1), but there are exceptions.
Figure 2. Models of seismic
S-wave velocity in the deep mantle. PREM is
an average Earth model. The other models, determined
for the localized regions shown on the map in
Figure 1 by analysis of seismic waves, all indicate
the presence of a 2-3% shear velocity discontinuity
200-300 km above the core mantle boundary (2891
km deep). This is well-explained by the presence
of post-perovskite in the lowermost mantle in
these regions.
|
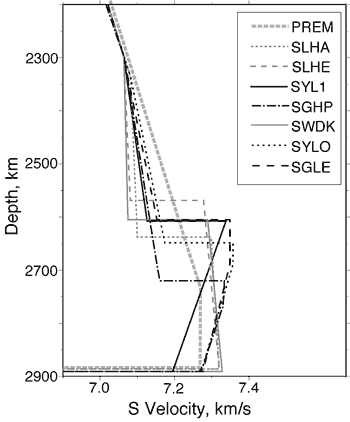
|
After decades of puzzling
over the nature of the D" discontinuity, a viable
explanation is now to hand. In 2004, mineral physics
experiments discovered that the predominant lower-mantle
mineral, magnesium-silicate perovskite [(Mg1-x,Fex)SiO3]
undergoes a transition to a post-perovskite polymorph
at temperatures and pressures similar to those in D"
(Murakami et al., 2004; Oganov & Ono,
2004; Iitaka et al., 2004). This phase change
is expected to result in a ~2% increase in S
velocity, a small decrease or increase in P
velocity, and a 1% density increase. Theoretical models
suggest a strong positive Clapeyron slope for the transition
(e.g., Tsuchiya et al., 2004), perhaps twice
as strong as that of the 410-km deep transition from
olivine to β-spinel (Wadsleyite). This is relevant to
the mantle plume hypothesis, because this phase change
would favor generation of thermal boundary layer instabilities
and vertical transport of material in the deep mantle
(Lay et al., 2005). The positive Clapeyron
slope and the predicted superadiabatic temperature increase
in D" could lead to post-perovskite transforming
back to perovskite at the very base of the mantle (Hernlund
et al., 2005) if the temperature increase is sufficient.
This would result in a velocity decrease there that
seismologists can seek, although it is much harder to
observe a decrease than the velocity increase from the
shallower phase boundary (e.g., Flores & Lay,
2005).
If the post-perovskite
phase does actually exist in the lowermost mantle, it
will occur in the lower-temperature regions of D".
This could account for the correlation between where
a shear-velocity discontinuity is observed and where
high velocity (lower temperature?) volumetric structure
is found in the boundary layer. Helmberger et al.
(2005) assume that tomographic shear-velocity variations
in D" are primarily a thermal effect, and they
map the variations into a topography map for the position
of the phase boundary; shallower in the mantle under
higher-velocity regions and deeper in the mantle under
lower-velocity regions (Figure 3). In order to account
for the large-scale pattern in the velocities, it is
often suggested that subducting oceanic lithosphere
has reached and ponded above the CMB, with sufficient
thermal anomaly retained to affect the seismic velocities
(e.g., Garnero & Lay, 2003; Sidorin
et al., 1999). Due to the lower temperature of
slab material compared to normal boundary-layer material,
one would then infer that the coldest regions of slab
material should have a thicker layer of post-perovskite
material. If ambient mantle temperatures are too high
for the post-perovskite phase to exist, it might even
be that any post-perovskite phase is confined to the
vicinity of recently delivered slab material that has
not yet thermally equilibrated. Lateral variations in
the depth of the D" discontinuity, including abrupt
steps in depth, have been interpreted as the result
of thermal variations within folded, ponded slab material
beneath downwellings, modulating the phase boundary
(e.g., Helmberger et al., 2005; Hutko et
al., 2006). Pretty speculative, but not implausible.
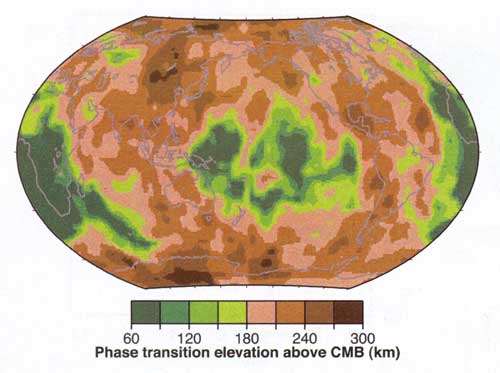
Figure 3. Mapping
of shear velocity heterogeneity into depth of
the perovskite to post-perovskite phase transition
relative to the CMB assuming a Clapeyron slope
of 6 MPa/K with reference height of 200 km above
the CMB and the tomographic model in Figure 1.
(From Helmberger et al., 2005). |
The large low-shear-velocity
provinces under the Pacific and Africa appear to have
a compositional contribution to their anomalous velocities
(e.g., Lay et al., 2004). The effects of iron
and aluminum on the post-perovskite phase are being
examined by experiments and theory, with iron likely
reducing the pressure at which the transition occurs
while aluminum increases the depth extent over which
it goes to completion. The post-perovskite phase appears
to have the capacity to take in surprisingly large amounts
of iron ((Mg1-x,Fex)SiO3
where x ~ 0.4, e.g., Mao et al., 2004), and
such strong iron enrichment can reduce seismic velocities
dramatically, possibly even to the extent needed to
account for the ultra-low velocity zones (ULVZ) detected
just above the CMB without requiring any partial melting
(W. Mao, personal communication, 2005). This later finding,
if substantiated, may have a big impact on our thinking
about the role of partial melting or melt components
in the ULVZ, and implications for thermal plumes as
well.
An important recent observation
is that a shear-velocity D" discontinuity about
230 km above the CMB is observed in the central Pacific
on the northern end of the large low-shear-velocity
province (Avants et al., 2006). As this region
(Figure 1) is far removed from any subduction zone and
has velocities lower than average in tomographic models,
attributing the discontinuity to the post-perovskite
phase transition requires that there be no direct connection
to deep slab material. This implies very widespread
occurrence of the shear-velocity discontinuity (and
the phase transition) along with possible elevation
of the discontinuity by increased iron content in a
region warmer than average (not allowed for in Figure
3). In this region, but not in circum-Pacific regions
(e.g., Flores & Lay, 2005), a deeper comparable-sized
velocity decrease is observed about 80 km above the
CMB. This could be a double discontinuity as postulated
by Hernlund et al. (2005). A confirmed double
crossing of the phase boundary would provide the best
direct measure of the superadiabatic thermal gradient
in D" yet obtained.
So, what does all this
mean for plumes coming from D"? As yet, the imaging
of hot upwelling features from D" remains very
controversial, but the possibility has not been ruled
out (see Banana Doughnut
page). The large-scale structure of D" leads most
geodynamicists to infer a major role at least for mid-mantle
convection in inducing the large-scale structure observed.
If D" is indeed dynamically circulating lower-mantle
material, the presence of the post-perovskite phase
is expected to enhance the vertical transport of material
and thermal-boundary-layer instabilities. Thus, recent
seismological developments can be inferred to strengthen
the hypothesis of large-scale thermal plumes rising
from the D" boundary layer, likely with diameter
scales of several hundred kilometers that should eventually
be resolvable by seismic tomography.
The main caveat remains
the uncertain nature of the chemical heterogeneity that
is believed to exist at least in the low-velocity regions
of Figure 1. If iron enrichment is involved, its effects
may compete with those of thermal buoyancy, mitigating
the tendency to develop boundary layer instabilities.
Superplumes, may simply be superpiles of iron-rich materials,
possibly relics of core formation or ancient subduction,
and high-velocity regions may simply be relatively cool
post-perovskite masses with no slabs involved. More
seismological research is needed to partition the effects
of thermal and chemical heterogeneity in D" and
to image better the small-scale heterogeneity if we
are to answer the question of whether plumes rise from
the lowermost mantle.
|
References
-
Avants, M., T. Lay,
S. A. Russell, and E. J. Garnero (2006). Shear-velocity
variation within the D" region beneath the
Central Pacific, J. Geophys. Res., in review.
-
Flores, C., and
T. Lay (2005). The trouble with seeing double, Geophys.
Res. Lett., Vol. 32, L24305,
doi:10.1029/2005GT024366.
-
Garnero, E. J.,
and T. Lay (2003). D" shear velocity heterogeneity,
anisotropy and discontinuity structure beneath the
Caribbean and Central America, Phys. Earth Planet.
Inter., 140, 219-242.
-
Grand, S. (2002).
Mantle shear-wave tomography and the fate of subducted
slabs, Phil. Trans. Roy. Soc. London (Ser. A),
3260, 2475-2491.
-
Helmberger, D. V.,
T. Lay, S. Ni, and M. Gurnis (2005). Deep mantle
structure and the post-perovskite phase transition,
Proc. Nat. Acad. Sci. USA, 10.10732/pnas.05023504102.
-
Hernlund, J. W.,
C. Thomas, and P. J. Tackley (2005), A doubling
of the post-perovskite phase boundary and structure
of the Earth’s lowermost mantle, Nature,
434, 882-886.
-
Hutko, A., T. Lay,
E. J. Garnero, and J. S. Revenaugh (2006). A folded
slab at the base of the mantle imaged by migration,
Nature, in review.
-
Iitaka, T., K. Hirose,
K. Kawamura, and M. Murakami (2004). The elasticity
of the MgSiO3 post-perovskite phase in the Earth's
lowermost mantle, Nature, 430,
442-445.
-
Lay,
T. (2005). The deep mantle thermo-chemical boundary
layer: the putative mantle plume source, in Plates,
Plumes, and Paradigms, G. R. Foulger, J. H.
Natland, D. C. Presnall, and D. L. Anderson, editors,
GSA Special Paper 388, pp. 193-205.
-
Lay, T., and E.
J. Garnero (2004). Core-mantle boundary structures
and processes, in The State of the Planet: Frontiers
and Challenges in Geophysics (R. S. J. Sparks
and C. J. Hawkesworth, editors), Geophysical Monograph
Series, 150, IUGG Volume 19, 25-41.
-
Lay, T., E. J. Garnero
and Q. Williams (2004). Partial melting in a thermo-chemical
boundary layer at the base of the mantle, Phys.
Earth Planet. Inter., 146,
441-467.
-
Lay, T., D. Heinz,
M. Ishii, S.-H. Shim, T. Tsuchiya, J. Tsuchiya,
R. Wentzcovich, and D. Yuen (2005). Multidisciplinary
impact of the lower mantle perovskite phase transition,
EOS, 86, pp. 1, 5.
-
Mao, W. L., G. Shen,
V. B. Prakapenka, Y. Meng, A. J. Campbell, D. L.
Heinz, J. Shu, R. J. Hemley, and H.-K.
-
Mao (2004). Ferromagnesian
postperovskite silicates in the D" layer, Proc.
National. Acad. Sci., 101,
15,867-15,869.
-
Murakami, M. K.
Hirose, K. Kawamura, N. Sata, and Y. Ohishi (2004)
Post-perovskite phase transition in MgSiO3,
Science, 304, 855-858.
-
Oganov, A. R., and
S. Ono (2004). Theoretical and experimental evidence
for a post-perovskite phase of MgSiO3
in Earth's D" layer, Nature, 430,
445-448.
-
Sidorin, I., M.
Gurnis, D. V. Helmberger (1999). Dynamics of a phase
change at the base of the mantle consistent with
seismological observations, J. Geophys. Res.,
104, 15,005-15023.
-
Tsuchiya, T., J.
Tsuchiya, K. Umemoto, and R. M. Wentzcovitch (2004).
Phase transition in MgSiO3 perovskite
in the earth's lower mantle, Earth Planet. Sci.
Lett., 224, 241-248.
Reprints/pdfs of all articles
by T. Lay are available upon request. Email
Thorne Lay |
last updated 8th
January, 2006 |
|
|