 |
Giant
Dikes: Patterns and Plate Tectonics
|
|
|
J.
Gregory McHone1, Don
L. Anderson2 &
Yuri
A. Fialko3
1Stones2Gems,
9 Dexters Lane, Grand Manan, NB E5G 3A6 Canada greg@earth2geologists.net
2Seismological
Laboratory, California Institute of Technology, Pasadena, CA 91125
dla@gps.caltech.edu
3Institute
of Geophysics and Planetary Physics, Scripps Institution of Oceanography,
University of California San Diego, La Jolla, CA 92093-0225
fialko@radar.ucsd.edu
|
INTRODUCTION
Giant dikes typically exceed 30 m in width and 100
km in length, with some examples over 100 m wide and 1,000 km long.
Dikes are self-induced magma-filled fractures, and they are the dominant
mechanism by which basaltic melts are transported through the lithosphere
and the crust. These spectacular intrusions are likely to have fed flood
basalts in large igneous provinces (LIPs), including provinces where
the surface basalts have been diminished or removed by erosion.
Although giant dikes can intermingle with denser swarms
of smaller dikes of similar composition (and probably similar origin),
others occur in sets of several to a few dozen extremely large quasi-linear
or co-linear intrusions, which may gently bend and converge/diverge
at low angles across many degrees of latitude. Tectonic controls on
the formation of giant dikes appear to be independent and different
from structures related to smaller dike swarms. Theoretical modeling
and field observations help us to understand the essential physics of
magma migration from its source to its final destination in the upper
lithosphere.
This discussion focuses on geographic patterns, magma
parameters, and associated physical features that provide evidence and
arguments about the origins of giant dikes. In particular, we describe
how giant dikes may be tectono-magmatic features of lithospheric plates,
not necessarily resulting from deep-mantle plumes.
|
THE FACTS
A major peculiarity of giant dike swarms and associated
flood basalts is an enormous supply of magma from an upper mantle source
to the Earth's crust. For example, a single dike of the Proterozoic Mackenzie
swarm in Canada likely carried a volume of magma equivalent to the 100
years-worth of melt production of the contemporary global mid-ocean ridge
system. The large volumes of magma, as well as the high magma fluxes (individual
giant dikes may have been emplaced on a time scale of weeks to months,
and the entire magmatic episodes might have occurred on a time scale on
the order of 105 years) imply a major thermal or compositional
(or both) perturbation in the upper mantle, or a perturbation in the lithospheric
stress field, over a relatively short time.
The inferred high rates of magma production require a
robust advection of hot material from the upper mantle and/or a storage
mechanism (ponding, underplating) plus a release mechanism (diking? stress
valve?). The delivery of melt from the mantle to the surface may not have
been a single-stage process, or a steady-state process. Thermo-mechanical
and chemical arguments alone do not constrain the depth from which the
hot/fertile material was supplied to the melting area (in or below the
thermal boundary layer (TBL) or the base of the lithosphere or in or below
the crust). It is not certain that the source region of LIPs is strongly
superheated. The smaller the holding tank (magma chamber, underplate,
magma pond) and the larger the host rock undercooling with respect to
the solidus is, the shorter the lifetime.
The association of giant dike swarms and flood basalts
with mantle plumes (originally proposed by Morgan, 1981) is based
on the observation that some flood basalt provinces seem to initiate hotspot
tracks (e.g., Duncan & Richards, 1991), and on isotopic
signatures of flood lavas (Carlson, 1991). However, many flood
basalts are not associated with volcanic chains and many volcanic chains
observed on the ocean floor have no associated LIPs or obvious plume heads.
In some cases, this may be explained by the removal of the initiating
LIPs by subduction.
On continents, most large igneous provinces (Continental
Flood Basalts – CFB) occur on or along suture zones adjacent to
cratons. When a continent splits, there is often (but not always) a
transient burst of magmatism. Steady-state mature ridges take some
time to form. Magmatic activity associated with rifting is often correlated
with pre-existing faults, transforms, fracture zones etc. (Sykes,
1978). The association of CFBs with Archons (cratonic roots of Archean
age), suture zones and pre-existing faults is much closer than it is
with time-progressive volcanic chains. Some CFB even occur during continental
convergence. There is a cause and effect issue here. Does a large,
hot, buoyant mass of magma from deep in the mantle cause the split,
or does continental breakup result from plate tectonic processes that
then allow the egress of accumulated magma? The occurrence of CFBs
during convergence (e.g., Deccan,
Keweenawan, Siberian, Alpine/European(?)) and during late stages of breakup
(the North Atlantic Tectonic Province) suggests that the association
of plumes and breakup is not universal and may incorrect. The
sequence usually assumed is first plume head, then extension, then
CFB, and lastly breakup of the continent. However, extension, ponding,
diking and breakup may precede LIP eruption, e.g., the
formation of the North Atlantic Igneous province signalled the completion
of the Atlantic opening, not the initiation (Ed: see also Iceland
and North Atlantic pages).
A seemingly radial pattern of giant dike swarms is often
attributed to uplifts caused by mantle plume heads (Ernst et al.,
1997), although the amplitude of the resulting uplift is insufficient
to accommodate large dike swarms (such as the Mackenzie swarm) by simple
magmatic fracturing. Given large magma volumes in the source region, and
high fluxes during dike emplacement, it is possible that a major fraction
of the observed dike thicknesses was facilitated by non-dilatant mechanisms
(e.g., via melting of the dike walls). It follows that the extensional
stress in the crust after the dike emplacement may have been larger than
prior to the dike emplacement. If so, the radial or fanning patterns of
large dike swarms might be due to a self-induced stress field. If a large-scale
uplift was centered over the magma source, its main role was probably
to provide a gravitational driving force for a long-range lateral magma
transport. (Ed: See also “Comments
on giant dikes” by Richard Ernst).
Large volumes of magma involved in the flood basalt events
pose several mechanical problems. If the giant dike swarms were fed from
an intermediate storage region in the crust, there is a problem of space
for a large (thousands to millions of cubic kilometers) shallow magma
chamber. If dikes ascended directly from a mantle source, and spread horizontally
upon reaching a level of neutral buoyancy, it is unclear how large volumes
of gravitationally unstable melt might have accumulated in the source
region. One possibility is that most of the melting takes place while
the lithosphere is under horizontal compression, so that the melt cannot
not readily escape via dikes.
Regional uplift due to upwelling mantle generates horizontal
compression in the lower lithosphere, which may result in magmatic underplating.
A subsequent sub-vertical transport of the ponded magmas may be possible
if the horizontal compression is relieved, e.g., by thermally
activated creep, gravitational collapse, or tectonic extension. A regional
uplift is not a pre-requisite for trapping of large volumes of melt at
the base of the lithosphere, which may, in principle be accomplished by
any process resulting in horizontal compression of the lower lithosphere. |
VOLUME IN CONTEXT
The volumes associated with giant dike swarms and LIPs
are indeed enormous but must be considered in context. The largest LIP
is the Ontong-Java Plateau. If the 20-km-thick ocean crust there was the
result of draining an area 3 times larger than the plateau (because of
focusing to the apex of the triple junction) and if 20% melting was involved,
then only a 30-km thickness of mantle need be involved. Normal mantle
geotherms are well above the solidus in the approximate depth interval
from 30 to 50 km depth. For the older giant dike swarms and LIPs, the
mantle will have been hotter. A basic question then is: Can magma draining
by porous flow to a structural or permeability trap in or beneath the
plate accumulate for a sufficient length of time to provide 106
km3 of magma in 106 years? Alternatively, can porous
flow in the mantle keep pace with the eruption rates? |
THE PARAMETERS
Parameters controlling dike intrusion include magma density
and viscosity, pressure in the magma, and stress magnitude and orientation
in the lithosphere. Yield stress in negligible compared to typical magmatic
overpressures, and host rock density affects propagation only insofar
as it affects the already-mentioned stress in the lithosphere. The tectono-magmatic
history of the area is also important. A long history of ponding (in the
lower crust or upper mantle) may be essential to the formation of long
diverging dikes. True radial dikes form as blade-like sheets of magma
that move laterally in the shallow crust. Simple gravitational stresses
on elevated features like Hawaiian volcanoes (Fiske & Jackson,
1971) or neutral buoyancy (Ryan, 1993) can control the depth
of lateral dike injection, but either way, the condition of formation
of radial dikes is that the difference between the intermediate and the
least principal stresses is less than the magma overpressure (the difference
between the magma pressure and the dike-perpendicular stress). No matter
what, true radial dikes are shallow, and the regions of lateral dike injection
rise, staying about the same depth below summits, as volcanoes grow.
Using the Hawaiian example, volcanoes with radial dike
configurations must stand in isolation and not nest against one another.
The volcanoes must be high enough that the region of dike injection is
not influenced by stresses in the ocean crust or upper mantle. If the
volcanoes nest, then rift zones with parallel dikes form. Bora Bora in
the Society Islands is an example of a high, freestanding volcano with
radial dikes. Isolated seamounts with stellate patterns of lateral rifts
(Vogt & Smoot, 1984) are another example. These are abundant in the
far western Pacific and formed when the Pacific plate was surrounded by
ridges and had minimal internal stress differences. Moreover, these are
swarms of “normal” dikes, not giant dikes.
Two of six dikes that radiate from Ship Rock volcanic neck in
New Mexico are shown in Figure 1. Ship Rock and its dikes were
probably only 750 to 1,000 m below the land surface at the time
they formed.
Figure 1: Dike radiating from Ship Rock. Photo: copyright
© Louis Maher, image courtesy Earth Science World ImageBank:
http://www.earthscienceworld.org/imagebank.
|
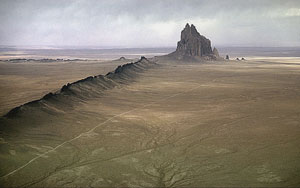
|
|
Length of dike
propagation is not an issue. The underlying stress
field is what controls whether dikes are radial
or parallel. Length has mainly to do with magma
supply and perhaps elevation of the source volcano
or magma chamber. Some Hawaiian rifts are hundreds
of kilometers long, as are dikes that originated
at some of the Hebridean intrusive centers. Those
are sub-parallel, cross much of Scotland and England,
and followed the direction of an aulacogen (the
abandoned third arm of a continental rift system).
These are examples where underlying lithospheric
stresses, not those of high volcanoes, influenced
dike orientation. Afar sits at the intersection
of three rift systems (Figure 2).It is not a radial
dike system, but a three-pronged “triple
junction”. Dikes may or may not be parallel
to the rift valley trends, but they are perpendicular
to rift extension directions. |
Rose diagrams and maps of dikes are needed to see how
truly radial any dike swarm may be. Some so-called radial
dike swarms are actually sub-parallel to or sub-perpendicular
to rifted margins. Unidirectional dikes from Iceland
have been proposed as the origin of the “V-shaped”
(chevron) bathymetric ridges that flank the Reykjanes
ridge. In other places (e.g., Scotland), later-generation
dikes cross-cut earlier dikes. In each case, the dikes
have been attributed to thermal plumes. (Ed:
See also “Comments
on giant dikes” by Richard Ernst).
A plume head could act to bulge up the crust and create
a broad region in which the intermediate and least principal stresses
become equal, even in the absence of a high volcano. More likely, however,
is that the stress configuration was that way to begin with. Some
diverging dike swarms, however, may have formed without a broad lithospheric
uplift. Uplift is not one of the requirements for radiating dikes. For
long-distance lateral injection, the main requirements are for a supply
of magma at either high pressure or elevated compared to the surroundings.
The surface itself need not be elevated, but the magma chamber needs to
be higher than the distant dikes which reach the surface (the artesian
effect), if diking is active, rather than just passive filling ofrifts.
If there is uplift and no topographic high, the dikes will track the level
of neutral buoyancy, which presumably is at the same level as the magma
chamber, and distant down-rift eruptions will be unlikely.
Mid-continent LIPs are places where large quantities
of melt accumulate beneath the lithosphere in blister-like masses that
may indeed cause uplift. Some uplift, combined with tectonic stress or
lithospheric weakening, may be the condition required before any magma
can escape. But when it does, the eruption rate is great and volume of
magma enormous. |
THE PHYSICS
A steady state between melting and erupting corresponding
to typical CFB fluxes (~1 km3/yr) is unlikely over geologic
time. At shorter time scales on the order of ~1 million years, such rates
of melt production could conceivably be attained given advection of hot,
fertile mantle material, as typically envisioned in the plume theory.
Problems arise if the timescale for melting is much greater than the timescale
for eruptive activity. Ponding is therefore essential for any theory,
and if ponding is allowed, a plume is unnecessary.
In the source region of flood basalts, there is obviously
a super- (or at least near-) solidus temperature. As melt is created,
it will immediately escape upward because it is gravitationally unstable.
Even a “regular” melting column (say, below a mid-ocean ridge)
does not contain more than a few percent of melt fraction. Given small
melt fractions, basalts may migrate upwards via porous flow in a viscous
matrix. The larger the melt fraction , the easier melt transport becomes.
If/when melt accumulates in larger bodies, upward transport occurs through
a much more efficient self-driven magma fracture (dikes). However, the
picture changes once magma leaves the source region and approaches the
sub-solidus (by some definitions) base of the lithosphere. In the lithosphere,
porous flow is impossible (melt freezes and clogs the pores), and must
be accomplished via dikes. Dikes must be thicker than a certain critical
width (~ 1 m) to avoid freezing. This implies that dikes must drain sizeable
pools of magma. Correspondingly, giant dikes must be fed from very
large reservoirs. Long-term existence of large reservoirs of melt at the
base of the lithosphere (e.g., in the case where horizontal compression
prevents diking) is unlikely, if that is where it ponds, because the melt
would exchange heat with the lower lithosphere and freeze. Assuming conductive
cooling alone, a 1-km-thick melt layer will lose most of its heat on a
time scale of 104 years.
However, the thermal boundary layer is not the same as
the lithosphere, which is defined by strength or viscosity and not by
thermal gradient. Melts can conceivably pond at density and permeability
interfaces, including in and below the region of conduction gradient.
In some models, the lower part of the thermal boundary layer can be of
the order of 1400°C. The lithosphere is defined by its long-term strength.
However, for silicate rocks strength is a function of temperature. An
upper mantle hotter than 900-1,000°C may not support any significant
deviatoric stress, and thus cannot create the compression required to
prevent vertical melt propagation. If melt ponds at a density interface,
it will not have any tendency for subsequent vertical propagation. Ponding
below a permeability barrier is probably the only option, similar to a
sub-axial magma lens at fast-spreading mid-ocean ridges. |
DO GIANT DIKE SWARMS
RADIATE?
Some of the longest Precambrian dike sets that exist
in northern Canada both curve and diverge, but diverging is not the same
as radiating. Radiating means extending out from a point in all directions,
not just one or two sets that spread out and bend along their great lengths.
Where are they on other sides of the focus points? And, why are there
not radiating dikes around all plume-uplift centers? Giant dikes
do not radiate around Iceland, Columbia River, Parana, Siberia, Deccan,
Yellowstone, or even Hawaii, although smaller dikes certainly do radiate
around some single volcanic centers (such as Ship Rock). The great Precambrian
dikes in Canada may have less to do with domal uplift than with extension
of the spherical shell of the lithosphere, which over a great distance
will not respond to stress like a flat planar surface. (Ed:
See also “Comments
on giant dikes” by Richard Ernst).
Late Archean – Early Proterozoic giant dike swarms
exposed in the Canadian Shield have a variety of geometries (Figure 3).
Dike sets may remain sub-parallel within great sweeping curves or branches
(the Matachewan dikes), or form sets of roughly parallel dikes (the Fort
Frances dikes), or diverge from a hypothetical focus point that they do
not actually reach (the Mackenzie and Mistassini dikes). Dikes far apart
and with different orientations may be similar in age (Figure 3). There
are no “hotspot tracks” – that is, no volcanic chains
progress from a focus suggested for any of the giant dike swarms.
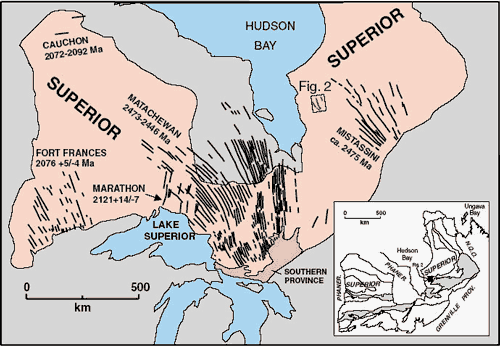
Figure 3: Late Archean – Early Proterozoic giant dike
swarms exposed in the Canadian Shield, from Hamilton et al. (2001).
|
One feature we may all agree on is that dikes form from
fluid magmas under pressure that follow (or create) propagating fractures,
which have orientations controlled by lithospheric stresses.
We suggest that if a set of parallel dikes propagates
far enough, the extensional fractures will curve and/or diverge because
they must follow the spreading, curving and domain-limited stress fields
of the spherical lithosphere. As the Earth's outer shell is pulled apart,
whether to form new rifts, drift the plates, or intrude dikes, there is
always a pole around which vectors of extension trace small circles.
The sub-parallel fractures must converge toward a pole and diverge away
from it. This will obviously not be apparent in sets of shorter dikes.
This is plate tectonics, not plume tectonics. |
PLATE MIGRATION VECTORS
AND DIKE PATTERNS
Linear directions followed by dikes are perpendicular to extension, but
they are also in planes that contain the direction of maximum compression.
Compression is created by plate collisions, but across the plate interiors
it is related to drag on the base of the lithosphere by the convecting
mantle. Dikes may form in the same directions as a consequence of plate
motions, as has been proposed for widespread Early Cretaceous dikes in
New England (McHone, 1988).
A map of absolute plate motion vectors in southern Asia, obtained
by very precise GPS measurements, has been recently presented
by Burchfiel (2004; Figure 3 therein reproduced with
permission herein as Figure 4). Because terranes converge and
rotate along the thrusts and suture zones of this region, motion
vectors are not parallel across the continent but instead form
sprays or fans within tectonic domains. These may also be directions
of maximum compression, analogous to the propagating fracture
planes of dikes. The patterns clearly have no relationship to
deep-mantle plumes impinging on the base of the lithosphere, although
dike swarms following these vectors might be called radiating.
(Ed: See also “Comments
on giant dikes” by Richard Ernst).
|
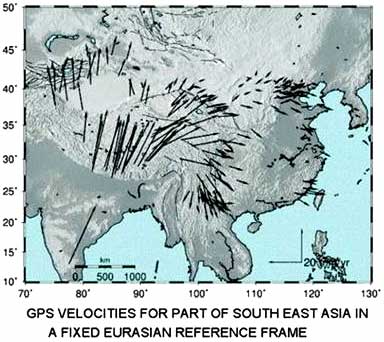
Figure 4: Absolute plate motion vectors in southern Asia,
from GPS measurements (Burchfiel, 2004). Reproduced with permission.
|
|
THE CENTRAL ATLANTIC
MAGMATIC PROVINCE
Fundamental observations by May (1971) first
suggested a radial pattern of dikes around the nascent rift zone of central
Pangaea, where the Atlantic Ocean crust would be initiated in Early Jurassic
time. This apparent pattern, with a focus somewhere east of Florida (perhaps
the Blake Plateau), has been used and re-used as evidence that a deep-mantle
plume produced the tholeiitic dikes and basalts, as well as continental
rifts of central Pangaea (e.g., Morgan 1971, 1981, 1983;
Ernst et al. 1995; Wilson 1997; Thompson 1998;
Ernst, Giant Radiating
Dyke Swarms, this website). Other studies have supported a non-plume
plate tectonic origin (e.g., Le Pichon & Fox 1971;
Sykes 1978; Lameyre et al. 1984; McHone 2000;
Hames et al. 2001). Much new work on this LIP, now called the
Central Atlantic Magmatic Province (CAMP), has
been published in a new monograph by Hames et al. (2002). Many
of the papers in that volume do not support a deep-mantle plume model
for the CAMP.
Cartoon maps of CAMP dikes reproduced
in plume-model papers have greatly exaggerated the radial geometry. This
distortion may be partly due to poor-quality maps for some areas of dikes
in Africa and South America. In fact, as discussed by McHone
(2000), the dikes occur in separate regions with distinct magma types,
and in many overlapping trend groups that do not radiate toward a common
point. Even the largest dikes within the regional groups do not physically
extend to a common focus at the Blake Plateau. In addition, most large
dikes tend to be discontinuous along strike, with en echelon
patterns or segments that are offset up to several kilometers. The magmas
of such dikes must interconnect in mainly vertical to oblique directions.
More commonly, swarms of many smaller dikes of the same magma type do
not interconnect in any lateral sense with dikes closer to the “plume
center.” The compositional correlations among dikes within groups,
the trend and compositional differences between groups, and the mainly
vertical flow required by dike structures, indicate that each dike group
is derived from its own mantle source, and that each group has its own
regional tectonic control.
Figure 5(a): The great N-S dike group that diverges from Charleston,
South Carolina northward into Virginia. From Hames et al. (2001). |
Figure 5(b): Cross-cutting of older high-Mg NW-SE dikes and
rift basins in North Carolina. From Olsen et al. (1991). |
However, like the giant dikes of northern Canada, some
of the very long dike systems in eastern North America do not remain parallel.
A great N-S group diverges from Charleston, South Carolina (not a “plume
center”) northward into Virginia (Figure 5a). This narrow dike set
cross-cuts older high-Mg NW-SE dikes and rift basins in North Carolina
(Figure 5b). These juxtaposed N-S and NW-SE dike sets are formed independently
and from different magmas (Ragland at al., 1983). The shorter
NW-SE dikes in the southern USA are numerous and occur in a very wide
belt from Virginia to Alabama (and beyond under the coastal plain), and
they remain sub-parallel from one end to the other because they are in
separate, short fractures rather than long, single ones.
In northeastern North America, huge but widespread dikes
in Canada and New England diverge to the NE and ENE from a focus point
east of New Jersey, but that is also not a plume center. The dikes change
their trends across the “New England Salient,” which is a
bend in terrane suture zones and primary structures of this section of
the Appalachian Orogen. In addition, the giant dikes did not form together
in a radial generation, but instead decrease systematically in age from
the SE toward the NW (Figure 6).
Figure 6: Map of NE North America showing the trend of dikes of
the “New England Salient” section of the Appalachian Orogen.
|
ANISOTROPY
OF MAGNETIC SUSCEPTABILITY (AMS) AND HORIZONTAL FLOW
Our last area of contention concerns the magmas of giant
dikes, which are proposed to flow horizontally away from plume centers,
possibly for hundreds or thousands of kilometers (Ed:
See also Giant radiating dyke swarms
page). Long-distance horizontal flow is required in this model, because
giant dikes are too large for vertical flow upward up from plume heads
over such great lengths. The evidence cited for horizontal flow is often
based on anisotropy of magnetic susceptibility (AMS), as measured from
oriented drill cores. Although their magmas follow propagating trans-crustal
fractures, the dikes themselves may not breach the surface along their
entire lengths because their magma density is relatively high.
The AMS of dikes can include a principal axis in a horizontal
plane, which has been used as definitive evidence for horizontal dike
flow (Tarling & Hrouda, 1993). AMS is mainly caused by small
magnetite grains, which crystallize late in the cooling history of basalts
(probably after magma flow has ceased). The magnetic anisotropy of basalt
is strongly controlled by plagioclase laths, around which the magnetite
grains collect in layers along the planar feldspar faces. In addition
to alignment by the primary magma flow, plagioclase crystals and resultant
AMS fabrics may become oriented by other mechanisms. As basaltic magmas
crystallize, a 3-D plagioclase network forms (Philpotts & Dickson,
2000), which eventually collapses and flattens if the magma body is large
enough (as in many sills and large dikes). A sub-horizontal preferred
crystal orientation results, which must affect the observed magnetic anisotropy
(usually a very subtle fabric).
Another cause can be back-flow after fluid pressure diminishes
in the later stages of dike activity. Magma movement back down into the
dike fractures has been observed in volcanic lava pools, and it could
re-orient the dike phenocrysts (commonly feldspars) and surrounding magnetite-rich
planes away from their initial upward or oblique directions.
Other features such as contact rip-ups, oriented phenocrysts
in glassy contact zones, wisps of melted country rock, and elongate contact
cusps can show initial dike flow directions, but even these may differ
from later, main-phase magma movements. AMS measurements by themselves
are not sufficient to demonstrate long-distance horizontal flow. |
SUMMARY
-
Huge volumes of tholeiitic magmas were generated
in upper-mantle source regions of giant dikes and may have caused
regional uplift or domal swells in the crust. Giant dikes propagated
fractures from these magma ponds in response to lithospheric tectonics,
with no requirement for deep-mantle plumes.
-
Non-radial patterns of fissure dikes are most
common for flood basalt provinces, not radiating giant dikes. True
radiating dikes are smaller and reflect local stress fields around
volcanoes or high-level magma chambers, not regional extension of
the lithosphere.
-
Giant Late Archean-Early Proterozoic dikes in
Canada are more accurately described as diverging or arcuate, not
radiating. None are connected to a progressive volcanic chain.
-
Very long sets of dikes do not remain parallel
but instead diverge as they propagate, because extensional stress
orientations vary across large distances in the spherical shell of
the lithosphere. There may be stress relationships with continental
rifting, plate divergence, mantle convection cells, and mid-ocean
ridges, but not “hot spot” plumes.
-
Giant dikes are not known beneath large surface
rifts, and radiating patterns of rifts on other planets are not related
to magmatic intrusions on Earth.
-
As shown by recent GPS motion vectors in southern
Asia, terranes can migrate and rotate along recent compressional sutures
or fault zones. Concordant dike swarms generated within tectonic domains
may have curving to sub-radial patterns because of variable stress
domains.
-
The giant dikes of the CAMP
are tectonic features clearly associated in geometry and location
with continental cratons and rift margins, and they occur in independent
but juxtaposed sets that are not radiating.
|
-
Carlson, R.W. 1991. Physical and chemical evidence
on the cause and source characteristics of flood basalt volcanism.
Austral. J. Earth Sci. 38, 525-544.
-
Burchfiel, B.C.. 2004. New Technology; New Geological
Challenges. GSA Today 14, 4–9.
-
Burke, K., 1976, Development of graben associated
with the initial ruptures of the Atlantic Ocean.Tectonophysics,
36, 93-112.
-
Burke, K.C. & Wilson, J.T. 1976. Hot spots
on the Earth’s surface. Sci. Am. 235,
46-57
-
Deckart, K., Féraud, G., Bertrand, H., 1997.
Age of Jurassic continental tholeiites of French Guyana/Surinam and
Guinea: Implications to the initial opening of the central Atlantic
Ocean. Earth Plan. Sci. Lett. 150, 205-220.
-
Duncan, R.A. and Richards, M.A., Hotspots, mantle
plumes, flood basalts, and true polar wander, Rev. Geophys.
29, 31-50, 1991.
-
Ernst, R.E., Head, W.J., Parfitt, E., Grosfils,
E., Wilson, L. 1995. Giant radiating dike swarms on Earth and Venus.
Earth Sci. Rev. 39,1-58.
-
Fiske, R.F, and Jackson, E.D., 1972. Orientation
and growth of Hawaiian volcanic rifts: the effect of regional structure
and gravitational stresses. Proc. Roy. Soc. (Lond.), A, 329,
299-326.
-
Gudmundsson, Agust. 2003. Surface stresses associated
with arrested dykes in rift zones. Bull. Volcanology 65,
606-619
-
Hames, W.E., Renne, P.R., Ruppel, C. 2000. New
evidence for geologically instantaneous emplacement of earliest Jurassic
Central Atlantic magmatic province basalts on the North American margin.
Geology 28, 859-862.
-
Hames, W.E., McHone, J.G., Ruppel, C., Renne, P.
(editors). 2002. The Central Atlantic Magmatic Province: Insights
from Fragments of Pangea: American Geophysical Union Monograph.
136, 267 pp.
-
Hamilton, M.A., Goutier, J., Mathews, W. 2001.
U-Pb baddeleyite age for the Paleoproterozoic Lac Esprit dyke swarm,
James Bay region, Quebec: Radiogenic age and isotopic studies. Report
14. Geological Survey of Canada, Current Research 2001-F5,
10 p.
-
Lameyre, J., Black, R., Bonin, B., Giret, A. 1984.
The magmatic provinces of eastern America, West Africa, and Kerguelen:
indications for a tectonic control of within-plate magmatism triggered
from above and associated processes. Ann. Soc. Geol. Nord
CIII, 101-114.
-
Le Pichon, X., Fox, P.J. 1971. Marginal offsets,
fracture zones, and the early opening of the North Atlantic. J.
Geophys. Res. 76, 6294-6308.
-
May, P.R.. 1971. Pattern of Triassic-Jurassic diabase
dikes around the North Atlantic in the context of the predrift positions
of the continents. Geol. Soc. Am. Bull. 82,
1285-1292.
-
McHone, J.G., 1988, Tectonic and paleostress patterns
of Mesozoic intrusions in eastern North America, in Manspeizer, W.R.,
ed., Triassic-Jurassic Rifting: Continental Breakup and the Origin
of the Atlantic Ocean and Passive Margins, Part B: New York,
Elsevier, 607-619.
-
McHone, J. G., 2000. Non-plume magmatism and tectonics
during the opening of the central Atlantic Ocean: Tectonophysics316,
287-296.
-
-
Morgan, W.J., 1981, Hotspot tracks and the opening
of the Atlantic and Indian oceans, in The Sea, 7,
443-487.
-
Morgan, W.J., 1983. Hotspot tracks and the early
rifting of the Atlantic. Tectonophysics 94,
123-139.
- Olsen, P.E., Froelich, A.J., Daniels, D.L., Smoot, J.P., Gore, P.J.W.
1991. Rift basins of Early Mesozoic age, in Horton, J.W. and Zullo,
V.A. (editors), Geology of the Carolinas. Knoxville, University
of Tennessee Press, 142-170.
-
Philpotts, A.R., Dickson, L.D., 2000. The formation
of plagioclase chains during convective transfer in basaltic magmas.
Nature 406, 59-61.
-
Ragland, P.C., Hatcher, R D., Jr., Whittington,
D., 1983. Juxtaposed Mesozoic diabase dike sets from the Carolinas.
Geology 11, 394-399.
-
Ryan, M.P., 1988. The mechanics and three-dimensional
internal structure of active magmatic systems: Kilauea volcano, Hawaii.
J. Geophys. Res. 93, 4213-4248.
-
Ryan, M.P., 1993. Neutral buoyancy and the structure
of mid-ocean ridge magma reservoirs. J. Geophys. Res. 98,
22,321-22,338.
-
Sykes, L.R. 1978. Intraplate seismicity, reactivation
of preexisting zones of weakness, alkaline magmatism, and other tectonism
post-dating continental fragmentation. Rev. Geophys. Space Phys.
16, 621-688.
-
Tarling, D.H., Hrouda, F. 1993. The Magnetic
Anisotropy of Rocks. Chapman and Hall, London, 217 pp.
-
Thompson, G.A., 1998. Deep mantle plumes and geoscience
vision. GSA Today, April, 17-25.
-
Vogt, P.R., 1973. Early events in the opening of
the North Atlantic. In: Tarling, D.H., Runcorn, S.K. (Editors), Implications
of Continental Rift to the Earth Sciences. Academic Press, New
York, 693-712.
- Vogt, P. R. and N. C. Smoot, The Geisha guyots: multibeam bathymetry
and morphometric interpretation, J. Geophys. Res. 89,
11,085-11,107, 1984.
-
Wilson, M., 1997. Thermal evolution of the Central
Atlantic passive margins: Continental break-up above a Mesozoic super-plume.
J. Geol. Soc. London 154, 491-495.
|
last updated March
2nd, 2004 |
|
|