 |
The
Source of Oceanic Volcanism – Plumes vs the
Low-Velocity Zone |
|
Dean
C. Presnall
Department
of Geosciences, University of Texas at Dallas,
Richardson, TX
presnall@utdallas.edu
This webpage is a summary of: Presnall,
D.C. and Gudfinnsson, G.H. (2011). Oceanic volcanism
from the low-velocity zone – without mantle plumes, Journal
of Petrology, Peter J. Wyllie Volume,
in press. (available on-line at Journal of
Petrology,
Advanced Access).
In a new model for oceanic
volcanism, Presnall & Gudfinnsson (2011) argue that oceanic volcanism originates from magmas
in the seismic low-velocity zone (LVZ) that ascend
through fractures in the seismic lithosphere. This
modeling is supported by:
- basalt glass compositions
from Hawaii and mid-ocean ridges;
- data on the melting
and crystallization behavior of natural lherzolite;
- phase
relations of model lherzolite in the systems CaO-MgO-Al2O3-SiO2-CO2 (CMAS-CO2)
and CaO-MgO-Al2O3-SiO2-Na2O-FeO
(CMASNF) at pressures from 1 atm to 6 GPa; and
- shear-wave velocities and anisotropy for the oceanic
upper mantle.
The LVZ, which extends from about
65 to 220 km depth in the ocean basins, was first suggested
to be a region of partial melting by Anderson
& Sammis (1970).
Wyllie & Huang (1975) and Eggler (1976)
then suggested that melting in the LVZ is caused by
CO2. This
argument has recently been reinforced (Presnall
& Gudfinnsson, 2005, 2011; Hirschmann,
2010). A
common assumption is that the melt fraction is very
small throughout the depth range of the low-velocity
zone. However, for a constant amount of CO2,
phase relations along a normal oceanic geotherm for
a lherzolite mineralogy in the CMAS-CO2 system
(Gudfinnsson & Presnall,
2005) indicate
a large tholeiitic melt fraction at ~ 4-5 GPa and smaller
alkalic (low-SiO2) melt fractions at both
lower (~ 3 GPa) and higher (> 6
GPa) pressures along the geotherm (Figure 2b in Presnall
& Gudfinnsson,
2011). Temperatures for
the model system are adjusted to those for natural
lhezolite on the basis of the volatile-free solidus
of garnet lherzolite at 5 GPa, 1500°C (Lesher et
al.,
2003) and the solidus for a natural carbonated lherzolite
(Dasgupta & Hirschmann, 2006). The
pressure range of Hawaiian tholeiitic melt-extraction
at ~ 4-5 GPa (~ 130-150 km depth) is based on the olivine-controlled
crystallization trend of Puna Ridge tholeiitic glasses
at Kilauea Volcano, Hawaii (Clague et
al.,
1995) combined with experimental data in the CMAS-CO2 system
(Gudfinnsson & Presnall,
2011, Figure
1).
In
the CMAS-CO2 system,
the melt-fraction at a given P, T, and bulk composition
in equilibrium with a lherzolite mineralogy is
a linear function of the amount of CO2 (Gudfinnsson
& Presnall,
2005, Figure 4). Because virtually all of
the CO2 is dissolved in the melt,
this would also be true for a natural carbonated
lherzolite. For the depth range where a geotherm
grazes the volatile-free solidus (Figures 2a,
b in Presnall & Gudfinnsson,
2011), tholeliites exist at significantly
enhanced melt-fractions. At both higher and lower
pressures, where the geotherm is at significantly
lower temperatures than the volatile-free solidus,
melt-fractions are much smaller. Thus, the equilibrium “magma
stratigraphy” in the LVZ along a normal
geotherm, as modeled by the CMAS-CO2 system,
would consist of small melt-fractions of alkalic
magma at shallow depths (~ 100 km), large melt-fractions
of tholeiitic magma at intermediate depths (~
120-170 km) and small melt-fractions of alkalic
magma at extreme depths (~190 km).
A southeastward-propagating
fracture system (Jackson et al.,
1972, 1975; Jackson
& Shaw, 1975) that extends into
the low-velocity zone and taps progressively
shallower magmas to the southeast would show
exactly the sequence and relative volumes
of magmas observed along the Hawaiian chain
as well as for individual Hawaiian volcanoes.
The depth of ~ 130-150 km for maximum melting,
as indicated by the phase relations (Gudfinnsson & Presnall,
2005), is consistent
with the depth of maximum shear-wave anisotropy
(Ekström, 2000) and lowest
vertical shear-wave velocity (Maggi et
al., 2006) throughout the central Pacific
Basin. The classical concept of decompression
melting (for example in a rising mantle plume)
is replaced by sampling of the equilibrium
magma-stratigraphy that exists in the Pacific
LVZ along a normal age-dependent geotherm.
At mid-ocean ridges, the range
of basalt glass compositions is controlled dominantly
by low-pressure crystallization of olivine, plagioclase,
clinopyroxene and orthopyroxene, as at Hawaii.
However, unlike Hawaii, no mid-ocean ridge basalt
(MORB) glass compositions, including those at Iceland,
exist that indicate more than an insignificant
amount of initial olivine-controlled crystallization
at low pressures. As the PetDB database used by Presnall
& Gudfinnsson (2011) is
very large (6,933 glass analyses), this is a robust
constraint. |
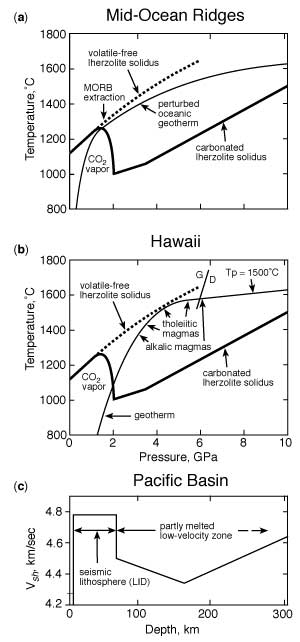
Figure 1. Comparison of horizontal
shear velocities (Vsh) for a SW-NE section across
the Pacific Basin (Tan & Helmberger, 2007)
(c) with solidus curves and geotherms for Hawaii
(b) and mid-ocean ridges (a). G/D is the graphite-diamond
transition (Bundy et al., 1961). The geotherm
for Hawaii assumes a 1500°C adiabat. The natural
volatile-free lherzolite solidus (bold dashed line)
is constrained at 5 GPa,1600°C by the determination
of Lesher et al. (2003). The natural carbonated
lherzolite solidus (bold continuous line) is from
Falloon & Green (1989)
and Dasgupta & Hirschmann (2006). Tp is
potential temperature. The P-T space between these
two curves is a region of olivine+orthopyroxene+
clinopyroxene+garnet+carbonate-bearing melt. The
slight change in slope of the carbonated lherzolite
solidus at 3.5 GPa is caused by intersection of
the solidus with the dolomite-magnesite transition
(Dalton & Presnall, 1998). |
Phase relations for the systems CaO-MgO-Al2O3-SiO2 and
CaO-MgO-Al2O3-SiO2-Na2O-FeO
(Presnall & Gudfinnsson,
2011, and references therein) show that MORB magmas,
including those at Iceland, are extracted at ~ 1240-1260°C,
1.2–1.5 GPa. Pressures of melt-extraction
higher than this would show olivine-controlled crystallization
paths like that found at Hawaii. Thus, melt-extraction
at mid-ocean ridges occurs at the shallow depth of
~ 65 km, the maximum pressure for melt-extraction without
olivine-controlled crystallization on subsequent cooling.
This depth is in excellent agreement with seismic data
(Nishimura & Forsyth, 1989) showing that
the depth range of minimum shear velocity (maximum
melt-fraction) at the crest of the East Pacific Rise
is ~ 40-70 km. These very low P-T conditions of melt-extraction
are within the thermal boundary layer, and indicate
a perturbed geotherm that grazes the lherzolite solidus
at these conditions (Figure 2, Presnall
& Gudfinnsson,
2011). Such a geotherm requires a steepened conductive dT/dP gradient
beneath ridges, which is consistent with heat-flow
data (e.g., DeLaughter
et al.,
2005).
Modeling
by Conder et al.
(2002) indicates eastward and upward flow within the
low-velocity zone beneath and just westward of the
East Pacific Rise. In agreement with this modeling,
a northwest to southeast cross-section of shear-wave
anisotropy across the Pacific Basin (Ekström,
2000) shows that the depth of maximum anisotropy is
fairly constant at ~130-150 km for the central Pacific
but shallows significantly as the East Pacific Rise
is approached from the northwest. Similarly,
Nishimura & Forsyth (1989) found that
the depth of minimum vertical shear velocity decreases
from ~150 km to ~ 40-70 km as the East Pacific Rise
is approached from the northwest. This essentially
perfect agreement of the seismic and phase equilibrium
data for the depths of maximum melting and tholeiitic
magma production at Hawaii and mid-ocean ridges is
powerful evidence that all oceanic volcanism comes
from fractures that tap melts in the low-velocity zone,
not from plumes.
References
-
Anderson, D.L. and Sammis, C.G.
(1970). Partial melting in the upper mantle, Physics
of the Earth and Planetary Interiors, 3,
41-50.
-
Bundy, F.P., Bovenkirk, H.P.,
Strong, H.M. & Wentorf,
R.H., Jr (1961). Diamond-graphite equilibrium
line from growth and graphitization of diamond. Journal
of Chemical Physics, 35,
383-391.
-
Clague, D.A., Moore, J.G., Dixon,
J.E. and Friesen, W.B. (1995). Petrology of submarine
lavas from Kilauea’s Puna Ridge, Hawaii. Journal
of Petrology, 36, 299-349.
-
Conder, J.A., Forsyth, D.W.
and Parmetier, E.M. (2002) Asthenospheric flow
and asymmetry of the East Pacific Rise, MELT area. Journal
of Geophysical Research, 107,
2344, d0i:10.1029/2001JB000607.
-
Dalton, J.A. & Presnall,
D.C. (1998). The continuum of primary carbonatitic-
kimberlitic melt compositions in equilibrium with
lherzolite: Data from the system CaO-MgO-Al2O3-SiO2-CO2 at
6 GPa. Journal of Petrology, 39,
1953-1964.
-
Dasgupta,
R. and Hirschmann, M.M. (2006). Melting in the
Earth’s
deep upper mantle caused by carbon dioxide. Nature, 440,
659-662.
-
DeLaughter,
J.E., Stein, C.A. and Stein, S. (2005). Hotspots:
a view from the swells, In:
Foulger, G.R., Natland, J.H., Presnall, D.C.
and Anderson, D.L. (eds.) Plates, Plumes,
and Paradigms, Geological Society of America
Spec. Paper 388, 257-278.
-
Eggler, D.H. (1976). Does CO2 cause
partial melting in the low-velocity layer of the
mantle?, Geology, 4,
69-72.
-
Ekström, G. (2000). Mapping
the lithosphere and asthenosphere with surface
waves: Lateral structure and anisotropy. In: Richards,
M.A., Gordon, R.G., and van der Hilst, R.D.
(eds.) The History and
Dynamics of Global Plate Motions. American
Geopysical Union, Geophysical Monograph 121,
239-254.
-
Falloon,T.J. & Green, D.H.
(1989). The solidus of carbonated, fertile peridotite. Earth
and Planetary Science Letters, 94,
364-370.
-
Gudfinnsson, G.H. and Presnall,
D.C. (2005). Continuous gradations among primary
carbonatitic, kimberlitic, melilititic, picritic,
and komatiitic melts in equilibrium with garnet
lherzolite at 3-8 GPa. Journal of Petrology, 46,
1645-1659.
-
Hirschmann, M.M. (2010). Partial
melt in the oceanic low velocity zone, Physics
of the Earth and Planetary Interiors, 179,
60-71.
-
Jackson,
E.D. and Shaw, H.R. (1975). Stress fields in
central portions of the Pacific plate: Delineation
in time by linear volcanic chains. Journal
of Geophysical Research, 80,
1861-1874.
-
Jackson,
E.D., Silver, E.A. and Dalrymple, G.B. (1972).
Hawaiian-Emperor chain and its relation to Cenozoic
circumpacific tectonics. Geological
Society of America Bulletin, 83,
601-618.
-
Jackson, E.D., Shaw, H.R.
and Bargar, K.E. (1975). Calculated geochronology
and stress field orientations along the Hawaiian
chain. Earth and
Planetary Science Letters, 26,
145-155.
-
Lesher, C.E., Pickering-Witter,
J., Baxter, G., and Walter, M. (2003). Melting
of garnet peridotite: Effects of capsules and thermocouples,
and implications for the high-pressure mantle solidus. American
Mineralogist, 88,
1181-1189.
-
Maggi, A., Debayle, E., Priestley,
K. and Barroul, G. (2006). Multimode surface waveform
tomography of the Pacific Ocean: a closer look
at the lithospheric cooling signature. Geophysical
Journal International, 166,
1384-1397.
-
Nishimura, C.E. and Forsyth,
D.W. (1989). The anisotropic structure of the
upper mantle in the Pacific, Geophysical
Journal, 96, 203-229.
-
Presnall,
D.C. and Gudfinnsson, G.H. (2005). Carbonate-rich
melts in the oceanic low-velocity zone and deep
mantle. In: Foulger, G.R., Natland, J.H., Presnall,
D.C. & Anderson,
D.L. (eds.) Plates, Plumes, and Paradigms.
Geological Society of America Special Paper 388,
207-216.
-
-
Tan,Y. & Helmberger, D.V.
(2007). Trans-Pacific upper mantle shear velocity. Journal of Geophysical
Research, 112, BO8301,
doi:10.1029/ 2006JB004853.
-
Wyllie,
P.J. and Huang, W.-L. (1975). Influence of mantle
CO2 in the generation of carbonatites
and kimbelites, Nature, 257,
297-299.
last updated 5th
May, 2011 |