 |
|
Primary
magmas at mid-ocean ridges, ‘hot-spots’ and other
intraplate settings: constraints on mantle potential temperatures
|
|
|
Most investigations of basalt petrogenesis define primary magmas as
those which have formed by partial melting within the upper mantle and
thus have chemical compositions (major element, trace element and isotopic
signatures) determined by:
-
Source composition i.e. the composition of the
bulk system experiencing partial melting. The source composition concept
includes the contents of volatile species particularly of the C-H-O
system (H2O, CO2, CH4), and
-
The P,T conditions of partial melting.
1. and 2. combine, in the time-scale of magmatic processes,
to produce crystalline phases, a melt phase, and possibly a fluid phase,
at equilibrium, i.e. the isotopic signature of the melt represents that
of the source and the trace-element, minor-element and major-element compositions
of the melt and all crystalline phases represent equilibrium partitioning.
This rationale allows us (for example) to use the Fe/Mg partitioning between
olivine and melt to screen natural glass compositions, or mantle xenolith-bearing
magma compositions, to infer that for the Earth’s mantle with olivine
Mg# ~90, primary magmas must have Mg# ~75. Similarly for the Earth’s
mantle with modal Ol > Opx > Cpx > Ga, Sp or Plag, a primary
magma must have some conditions of P,T, and activities of C-H-O fluid
species, where it is multiply saturated with olivine, orthopyroxene and
possibly spinel, clinopyroxene, and garnet or plagioclase. |
This rationale has led to the two-pronged investigation
of basalt petrogenesis by high-pressure experimental methods in which
both the progressive melting behaviour of peridotite + (C-H-O) is investigated
(defining compositions of residual phases and, under optimal conditions,
of the melt phase also) and the liquidus phases of potential primary
magmas are determined (e.g., for the suite of mantle xenolith-bearing
magmas from alkali olivine basalts to kimberlites). The experimental
method is obviously directly applicable to batch melting or melt extraction
from upwelling diapirs but it is equally important for concepts of porous
melt flow and melt pooling since melt compositions in porous flow are
controlled by local compositions and P, T conditions. Melt increments
reflect local phase compositions and P,T conditions and pooled melt
likewise equilibrates (or attempts to) with wall-rock mineralogy, i.e.
a new bulk composition at the P,T conditions of the postulated melt
aggregation depth.
Just as the rationale above guides the identification
and understanding of the genesis of primary magmas, so it may be used
to identify and investigate evolved or derivative magmas formed by cooling
and crystal fractionation at low pressure (sub-volcanic magma chamber
processes) or at high pressures (mantle wall-rock reaction and precipitation,
mantle magma chambers). The latter processes explain examples of evolved
nepheline mugearites, and even phonolites in intraplate settings, which
contain high-pressure mantle xenoliths. The same data on crystal/liquid
equilibrium identify xenocrysts vs. phenocrysts, and evidence for magma
mixing in channels or magma chambers.
|
The melting regime for mantle-derived basaltic magmas
from intraplate settings (kimberlites, olivine melilitites, olivine
nephelinites, basanites, alkali-olivine basalts) requires the presence
of carbon and hydrogen (dissolved (CO3 = ) and (OH-) in the
melt phase. Magma genesis in intraplate settings can be understood in
terms of the lherzolite + (C-H-O) system. Magmas are derived from an
“incipient melting” regime which lies at temperatures below
the volatile-free lherzolite solidus, marking entry to the “major
melting” regime. Experimental petrology and observations of natural
magmas concur in defining magma segregation at deep levels and magma
transport within channels with only limited wall-rock reaction. In the
intraplate settings, the role of an “incipient melting”
field in lherzolite + (C-H-O) at depths of > 90 km to ?< 200 km
creates conditions in which both depletion (from N-MORB to D-MORB sources)
and enrichment (from N-MORB to E-MORB sources) occur by migration of
a 1-2 % melt fraction.
These relationships are summarized in a specific model
for a petrological lithosphere (below the silicate solidus for lherzolite
+(C-H-O)) and asthenosphere (above the silicate solidus) (Figure 1)
The proposed model is used to explain production of specific primary
magmas in the P,T field between the regionally applicable conductive
geotherm and adiabatic upwelling of mantle with potential temperature
(Tp) ~1430°C (Figure 2).
|
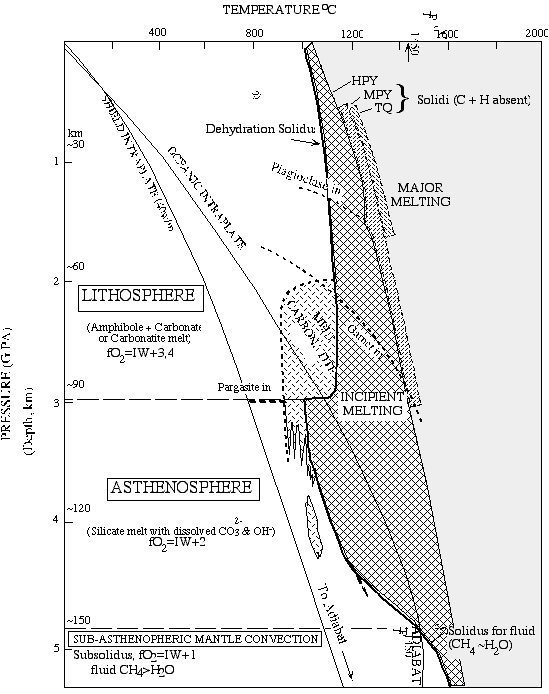
Figure 1: Application of the experimentally determined
melting relationships for pyrolite and pyrolite+(C+H+O)
to model the intraplate lithosphere, asthenosphere
and subasthenospheric mantle. The ‘oceanic intraplate’
geotherm represents a geothermal gradient appropriate
to old oceanic crust and ‘young’ continental
crust. This geotherm necessarily traverses the carbonatite
stability field and incipient melting regime for the
pyrolite compositions provided mantle fO2≥IW+2
log units. Attention is drawn to the petrological
base of the lithosphere defined by the high pressure
pargasite breakdown and silicate solidus at ~95 km.
The lower boundary of the asthenosphere (incipient
melting regime along the geotherm) is drawn arbitrarily
at ~150 km as the intersection of a mantle adiabat
of potential temperature Tp=1450°C with the pyrolite-(C+H+O)
solidus for fO2 = IW+1. Below this depth,
a fluid with CH4 ≥ H2O
is present but there is no silicate melt unless fO2
> IW+1, i.e. a more oxidised region of the mantle.
The carbonatite melt at > 95 km and T < 1000°C
is represented as present or absent dependent on local
variation in fO2 i.e. if fO2 ≥
IW+3 then carbonatite melt ± H2O-fluid
(rather than graphite (diamond)+H2O-fluid)
would be present.
|
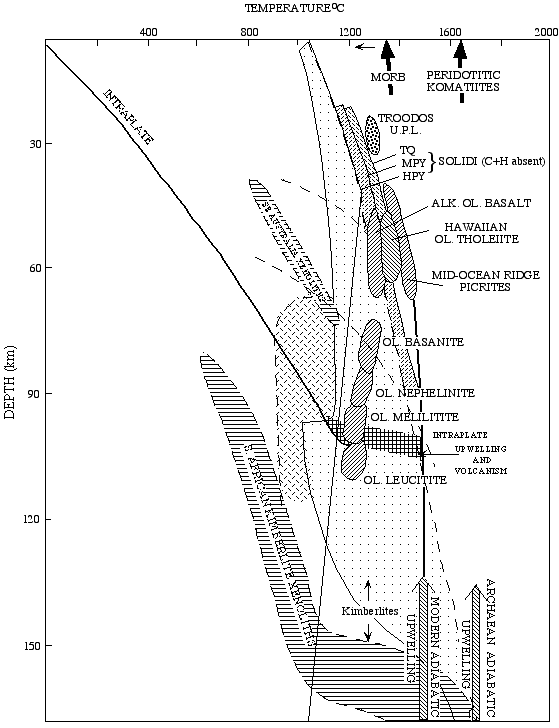
Figure 2: The basis for the view that the modern
mantle adiabat has a potential temperature of ~1450°C
and all modern volcanism, whether hot-spot, back-arc
or intraplate rifting, can be understood within the
temperature envelope between the appropriate geotherm
for the thermal boundary layer and the mantle adiabat
(Tpa1450°C)
Attention is also drawn to the inference that Archaean
peridotitic komatiites imply mantle potential temperatures of at least
1650°C.
Also illustrated is the P,T field inferred for
spinel lherzolite, pyroxenite and garnet pyroxenite xenoliths in the
Newer Volcanics of southeast Australia. This is considered to represent
a perturbed lithosphere geotherm during intraplate, rift-related volcanism
and mantle metasomatism in southeast Australia. The field for South
African kimberlite xenoliths has two components — the field enclosing
the 40W/m2 geotherm (Figure 2) and the high temperature ‘kink’
of enigmatic origin. In this figure, this region, and a similar kink
at the lower end of the intraplate geotherm, are represented as transient
phenomena associated with adiabatic upwelling and magmatism linked with
lithospheric plate movement and thinning.
|
In many Earth models, mid-ocean ridge (MOR) magmatism
is attributed to decompression melting of upwelling upper mantle/asthenosphere
at normal mantle temperature and melting has been considered to occur
in the absence of significant volatiles (C-H-O). Nevertheless, primitive
MORBs have water and carbon contents which are not negligible but rather
are very low because of relatively high degrees of melting of a source
with low carbon and hydrogen contents. Most importantly, primitive MORBs
have remarkably restricted compositions compatible with saturation with
olivine, orthopyroxene, minor clinopyroxene and Cr-Al spinel at pressures
around 2 GPa. Processes of melt migration by porous flow may have occurred
at deeper levels but at 2 GPa equilibrium between melt and lherzolite
mineralogy is indicated. Primary magmas move from this depth through dykes
or channels, without significant modification by wall-rock reaction, to
sub-ridge magma chambers or sea-floor eruption.
In the ‘hot-spot’
setting, combinations of geophysical arguments (the buoyancy implication
beneath the Hawaiian swell) and geochemical arguments (thicker crust,
‘garnet signature’ on REE implying
deeper melting if the anhydrous solidus is used) have been used to infer
higher temperature in the mantle, in comparison with the MOR setting.
The comparison of magma and phenocryst compositions between MOR and Hawaiian
primitive basalts is a way of testing for evidence for such temperature
contrasts, argued in some models to be of the order of 200°C. |
The composition of olivine phenocrysts in Hawaiian picrites and in
MOR picrites varies up to Mg# 91.3 and Mg# 92.1 respectively. The compositions
and liquidus temperatures of the magmas crystallizing the most magnesian
phenocrysts can be estimated and anhydrous liquids temperatures (at
1 bar pressure) of Hawaiian tholeiitic picrites average 1365°C,
and for MOR picrites average 1335°C. Water contents of the magmas
decrease in the order Hawaiian picrites, E-MOR picrites to N-MOR picrites,
and consideration of liquidus depression by these water contents leads
to the conclusion that all primitive magmas had liquidus temperatures
of approximately 1325°C at ~ 1 bar. The data from primitive magmas
suggests that the temperature contrast between “hot-spot”
and MOR primary magmas is ≤ 20°C. Application of information
from partial melting studies of model (pyrolite) source compositions
and of the liquidus phases of the hot-spot and MOR picrites leads to
the conclusion that both ‘hot-spot‘ and MOR primary basalts
are derived from mantle with potential temperature Tp ~ 1430°C.
Insofar as primitive magmas may be used to infer the potential temperature
of their sources, there is no evidence for a temperature contrast of
Δ Tp = 100-250°C between ‘hot-spot’
or ‘deep mantle plume’ sources
and ambient (MOR source) asthenospheric mantle.
Although magma temperatures are similar, the residual mantle compositions
for Hawaiian picrites are refractory harzburgites, more refractory (including
Cr/Cr+Al ratio) than the lherzolite to harzburgite residue from MOR
picrite extraction. It is argued that the buoyancy plume and geophysically
anomalous mantle beneath the Hawaiian swell is due to compositional
and not temperature contrasts in the upper mantle. The four-component
mixing identified in the Hawaiian source is attributed to interaction
between old subducted lithospheric slabs, buoyant or suspended in the
upper mantle, and surrounding ambient mantle at Tp =1430°C. A cartoon
representing the model is shown in Figure 3.
|
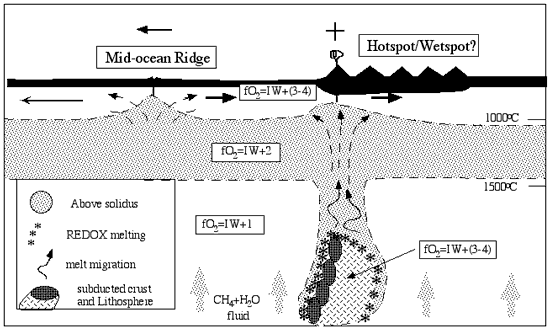
Figure 3: Cartoon suggesting a model for intraplate
‘hot–spot’ volcanism attributing
the primary cause of the melting anomaly (‘hot–spot’)
to a compositional anomaly within the mantle beneath
the asthenosphere, i.e. > 150–200 km depth.
Increased (C+H+O) volatile flux is attributed to redox
melting at the interface of ambient mantle with CH4+H2O
fluid (fO2=IW+1) and old subducted slab
or delaminated cratonic lithosphere (fO2=IW+3,4).
The geochemical anomaly (‘plume source’)
includes deep mantle (CH4+H2O
fluid), sub-asthenospheric and asthenospheric mantle,
and old, subducted sources in a mixing and upwelling
process. The geophysical anomaly (e.g. ‘Hawaiian
Swell’) is attributed to relative densities
of subducted slab/delaminated lithosphere and ambient
mantle, to the melt-enhanced column above the compositional
anomaly, and the thermal anomaly and thermal erosion
of the lithosphere by diapirism from >150 to 200
km depths leading to magma segregation within lithospheric
depths (40-60 km) (from Green & Falloon, 1998).
|
-
-
Green, D. H. and R. C. Liebermann (1976). Phase
equilibria and elastic properties of a pyrolite model for the oceanic
upper mantle. Tectonophysics 32: 61-92.
-
Green, D. H., T. J. Falloon, et al. (1987). Mantle-derived
magmas - roles of variable source peridotite and variable C-H-O fluid
compositions. Magmatic Processes and Physicochemical Principles, Geochem.
Soc. Spec. Publ. 12: 139-154.
-
Green, D. H. and T. J. Falloon (1998). Pyrolite:
A Ringwood concept and its current expression. pp 311-380 in The
Earth’s Mantle; Composition, Structure, and Evolution,
ed I.N.S. Jackson, Cambridge, Cambridge University Press, 566 pp.
-
Green, D. H., T. J. Falloon, S.M. Eggins and G.M.
Yaxley. (2001). Primary magmas and mantle temperatures. European
J. Min. 13: 437-451.
updated
1st January, 2007 |
|
|