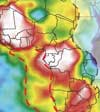 |
Mid-Lithospheric Discontinuity and a new hypothesis for oceanic plateau genesis |
Zhensheng Wang1, Timothy M. Kusky1,2, Fabio A. Capitanio1,3
1State Key Lab of Geological Processes and Mineral Resources, Center for Global Tectonics, School of Earth Sciences, China University of Geosciences, Wuhan, China; jasonwang@cug.edu.cn ; tkusky@gmail.com
2Three Gorges Research Center for Geohazards, China University of Geosciences, Wuhan, China
3School of Earth, Atmosphere and Environment, Monash University, Clayton, 3800 VIC, Australia, fabio.capitanio@monash.edu
This webpage is a summary of: Wang, Z., Kusky, T., Capitanio, F., 2017. Ancient continental lithosphere dislocated beneath ocean basins along the mid-lithosphere discontinuity: a hypothesis. Geophysical Research Letters, 44(18), 9253-9260.
Modern ocean basins are typically floored by lithosphere formed at spreading ridges or near mantle plume heads. The age of the oceanic lithosphere increases away from spreading centers to continental margins, where modern oceanic lithosphere is no older than 300 Ma [Müller et al., 2008]. The thickness of most of the oceanic lithosphere increases with the square root of its age until ~ 100 km [Doin & Fleitout, 1996]. High seismic-velocity anomalies are found beneath some oceanic basins, indicating that the lithosphere extends to greater depths in these places [e.g., King & Ritsema, 2000; Deen et al., 2006; Begg et al., 2009]. An example is the presence of thick lithosphere beneath the southern Atlantic margin adjacent to Africa where several studies reveal high shear-wave velocities (2-7%) at depths between 100-175 km or deeper [e.g., King & Ritsema, 2000; Deen et al., 2006; Begg et al., 2009] (Figure 1). This anomaly is laterally continuous with high-velocity anomalies in the cratonic area adjacent to it where the anomaly is ~8%. It is also found 300-1300 km away from the passive margin. Some of these areas may be continental lithospheric roots, possibly ancient cratonic lithosphere of Proterozoic or Archean ages (>1000 Ma) [Bonatti, 1990; Chazot et al., 2005; Deen et al., 2006; Begg et al., 2009; Coltorti et al., 2010] which were dislocated and embedded beneath oceanic basins 300-1300 km away from the passive margin [Bonatti, 1990; Chazot et al., 2005; Deen et al., 2006; Begg et al., 2009; Coltorti et al., 2010; Huismans & Beaumont, 2011].
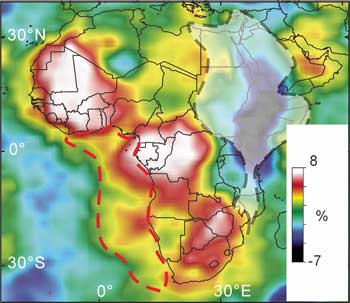
Figure 1: Seismic anomalies at 100-175 km depth near Africa as percent deviation from the 4.5 km/s average Vs at this depth (after Begg et al., 2009). The red dashed line outlines the oceanic region underlain by lithosphere with high shear-wave speeds that may correspond to displaced continental lithosphere.
This geophysically detectable, atypically thick, old lithosphere is distinct from regions that yield ancient magmatic components derived from heterogeneous mantle sources contaminated with ancient lithospheric fragments [Harvey et al., 2006; Liu et al., 2008; Hamelin et al., 2013]. It is also distinct from hyper-extended continental margins [Brune et al., 2014] where continental crust can be trapped in the newly forming oceanic lithosphere during stretching. These observations suggest that some parts of cratonic mantle lithosphere may not be permanently attached to the drifting continental lithosphere as previously thought, potentially highlighting a novel aspect of continent stability [Artemieva & Mooney, 2002; Begg et al., 2009; Huismans & Beaumont, 2011; Beaumont & Ings, 2012; Huismans & Beaumont, 2014; Kaban et al., 2015].
Why such volumes of what appears to be thick continental cratonic lithosphere are found beneath oceans remains controversial. Small-scale-convection-related down-welling may be involved [e.g., King & Ritsema, 2000]. Transport of buoyant cratonic root in continental interiors may result from strong basal drag beneath thick (>300 km) cratonic roots [Artemieva & Mooney, 2002; Kaban et al., 2015]. Buoyant cratonic roots may be dislocated with relatively small offsets (<100 km) by forces arising from lateral pressure gradients during the continental breakup [Huismans & Beaumont, 2011; Beaumont & Ings, 2012; Huismans & Beaumont, 2014]. Fragmentation and lateral disruption of the cratonic lithosphere may also occur along weak zones during rifting [Begg et al., 2009; O'Reilly et al., 2009].
Recently, a Mid-Lithospheric Discontinuity (MLD) has been detected at depths of ~60 to ~160 km, and generally between ~80 and 100 km, beneath most cratonic areas. It is characterised by low seismic velocities and a distinct seismic anisotropy signature [Thybo & Perchuć, 1997; Yuan & Romanowicz, 2010; Wölbern et al., 2012; Sodoudi et al., 2013; Selway et al., 2015; Calò et al., 2016; Aulbach et al., 2017]. Seismic velocity reductions across this layer are large (3–10%) and exceed those measured across the lithosphere-asthenosphere boundary (LAB) in continental areas which are ~1% or slightly larger [Selway et al., 2015 and references therein; Aulbach et al., 2017]. The fast-axis direction of azimuthal anisotropy in some cratonic areas (e.g., North America) changes sharply at MLD depths [Yuan & Romanowicz, 2010]. Proposed models for the MLD include enhanced partial melting or grain boundary sliding, enrichment in infiltrated frozen melts, pyroxenes, phlogopite, amphibole or carbonates, layering of minerals with distinct orientation, and changes in deformation creep mechanisms (boundary between diffusion- and dislocation creep) [Selway et al., 2015; Fei et al., 2016; Aulbach et al., 2017]. Some MLD’s may be weak layers in the mid-lithosphere [e.g., Thybo & Perchuć, 1997; Liao et al., 2013; Liao & Gerya, 2014 and references therein] where decoupling and shearing between the upper and lower part of the cratonic lithosphere can occur [Liao et al., 2013; Liao & Gerya, 2014].
The existence of the MLD is well-documented in the Tanzania and Kalahari Cratons of Africa. There the cratonic roots are inferred from deep-sourced xenolith compositions to be buoyant [Poudjom Djomani et al., 2001]. The MLD is possibly related to melt infiltration and metasomatism [Wölbern et al., 2012; Sodoudi et al., 2013] which can lead to localized MLD weakness and shearing.
We postulate that the MLD in the African cratons is a weak layer that locally underwent strong shearing and decoupling the cratonic lithospheric root thus allowing it to be laterally offset underneath the Atlantic oceanic basin during motion of the African plate (Figure 1). We investigate the viability of this mechanism using numerical modeling and find that large lithospheric offsets beneath the continent-ocean transition zone can be generated by shearing along a weak MLD during plate motion. Our results are consistent with geological examples and have broad implications for understanding the origin of poorly understood, thick, ancient lithosphere beneath oceanic basins [for modeling details see Wang et al., 2017].
The process proposed and modeled here can be divided into three stages. In the initial stage (Stage 0, Figure 2a), cratonic lithosphere with a weak MLD is stable because of insufficient weakness of the MLD, a large distance from a continental margin, or protection by surrounding orogenic belts. The lithosphere at this stage is likely similar to those of the present Superior, Slave, Yilgarn and western North China Cratons [Chen et al., 2014; Selway et al., 2015; Aulbach et al., 2017]. There, MLDs are imaged within the cratons, but are probably not weak enough to fail and are protected by surrounding orogenic belts [e.g., Lenardic et al., 2000] so the lithospheric roots are not offset beneath oceans. For cratons with sufficiently weak MLDs (low viscosity and large thickness) their roots would displace laterally during on-craton rifting [Liao et al., 2013; Liao & Gerya, 2014].
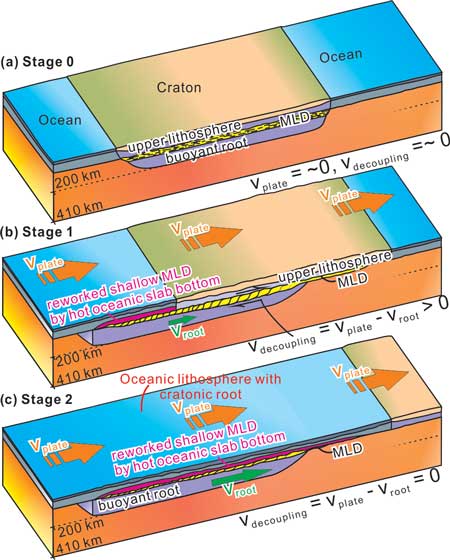
Figure 2: Simplified sketch showing decoupling and lateral offset of the buoyant cratonic root beneath oceanic basins along the MLD.
In the following stage (Stage 1), the upper lithosphere moves rapidly during plate motion, leaving the buoyant cratonic root behind beneath the oceanic-continent transition along the weakly coupled MLD (Figure 2b). The best example for this stage is likely the lithosphere near the SW Africa margin of the Atlantic. This process can be sustained by high plate velocities that drive progressive shear. Progressive thinning of the MLD results in increased coupling (Figure 2c) so the displaced buoyant cratonic root becomes anchored beneath, and coupled to, the overhead moving oceanic plate (Stage 2). This results in a new, hybrid oceanic/continental lithosphere with the upper section formed at an ocean ridge and cratonic root trapped beneath it. On emplacement in the oceanic realm, where temperatures are higher, the MLD heats and may melt. Melting in the lithospheric layers rich in amphibole, phlogopite, solidified melt, or volatiles [Griffin et al., 2003; Thybo & Perchuć, 1997; Foley, 2008; Wölbern et al., 2012; Selway, 2015] can occur, followed by upward migration of melt and intrusion into a new or reworked MLD beneath the ocean and above the original MLD.
A likely example of this stage is the layered lithosphere of the Ontong Java oceanic plateau. There, lower lithosphere with ancient continental features (~95-120 km or ~280 km thickness, aged 0.9-1.7 Ga) [Ishikawa et al., 2011; Tharimena et al., 2016] underlies the young oceanic lithosphere (<85 km thickness, aged <160 Ma) with a clearly detected ‘MLD’ (in the depth interval 40-80 km) in between [Ishikawa et al., 2011; Tharimena et al., 2016]. Although a proposed origin of this plateau is plume impingement [Ishikawa et al., 2011; Tharimena et al., 2016] this structure is also compatible with Stage 2 in our model. Additionally, blocks of ancient continental root underlying oceanic basins in the Atlantic [Bonatti, 1990; Coltorti et al., 2010] may also be examples of Stage 2. The model proposed here suggests that some ocean rises result from underplating by ancient, buoyant, compositionally light material from a strongly heterogeneous mantle [Dick & Zhou, 2014; Liu et al., 2008].
References
-
Artemieva, I. M., and W. D. Mooney (2002), On the relations between cratonic lithosphere thickness, plate motions, and basal drag, Tectonophysics, 358, 211-231.
-
Aulbach, S., M. Massuyeau, and F. Gaillard (2017), Origins of cratonic mantle discontinuities: A view from petrology, geochemistry and thermodynamic models, Lithos, 268, 364-382.
-
Beaumont, C., and S. J. Ings (2012), Effect of depleted continental lithosphere counterflow and inherited crustal weakness on rifting of the continental lithosphere: general results, J. Geophys. Res., 117, 10-1029.
-
Begg, G. C., W. L. Griffin, L. M. Natapov, S. Y. O'Reilly, S. P. Grand, C. J. O'Neill, J. Hronsky, Y. P. Djomani, C. J. Swain, and T. Deen (2009), The lithospheric architecture of Africa: Seismic tomography, mantle petrology, and tectonic evolution, Geosphere, 5, 23-50.
-
Bonatti, E. (1990), Subcontinental mantle exposed in the Atlantic Ocean on St Peter-Paul islets, Nature, 345, 800-802.
-
Brune, S., C. Heine, M. Pérezgussinyé, and S. V. Sobolev (2014), Rift migration explains continental margin asymmetry and crustal hyper-extension, Nat. Commun., 5, doi:10.1038/ncomms5014
-
Calò, M., T. Bodin, and B. Romanowicz (2016), Layered structure in the upper mantle across North America from joint inversion of long and short period seismic data, Earth Planet. Sci. Lett., 449, 164-175.
-
Chazot, G., S. Charpentier, J. Kornprobst, R. Vannucci, and B. Luais (2005), Lithospheric Mantle Evolution during Continental Break-Up: The West Iberia Non-Volcanic Passive Margin, J Petrol., 46, 2527-2568.
-
Chen, L., M. Jiang, J. Yang, Z. Wei, C. Liu, and Y. Ling (2014), Presence of an intralithospheric discontinuity in the central and western North China Craton: Implications for destruction of the craton, Geology, 42, 223-226.
-
Coltorti, M., C. Bonadiman, S. Y. O'Reilly, W. L. Griffin, and N. J. Pearson (2010), Buoyant ancient continental mantle embedded in oceanic lithosphere (Sal Island, Cape Verde Archipelago), Lithos, 120, 223-233.
-
Deen, T. J., W. L. Griffin, G. Begg, S. Y. O'Reilly, L. M. Natapov, and J. Hronsky (2006), Thermal and compositional structure of the subcontinental lithospheric mantle: Derivation from shear wave seismic tomography, Geochem. Geophys. Geosyst., 7, 329-349.
-
Dick, H.J.B. and H. Zhou (2014), Ocean rises are products of variable mantle composition, temperature and focused melting, Nat. Geo., 8, 68-74.
-
Doin, M. P., and L. Fleitout (1996), Thermal evolution of the oceanic lithosphere: an alternative view, Earth Planet. Sci. Lett., 142, 121-136.
-
Fei, H., S. Koizumi, N. Sakamoto, M. Hashiguchi, H. Yurimoto, K. Marquardt, N. Miyajima, D. Yamazaki, and T. Katsura (2016), New constraints on upper mantle creep mechanism inferred from silicon grain-boundary diffusion rates, Earth Planet. Sci. Lett., 433, 350-359.
-
Foley, S. F. (2008), Rejuvenation and erosion of the cratonic lithosphere, Nat. Geosci., 1, 503-510.
-
Griffin, W. L., S. Y. O'Reilly, L. M. Natapov, and C. G. Ryan (2003), The evolution of lithospheric mantle beneath the Kalahari Craton and its margins, Lithos, 71, 215-241.
-
Hamelin, C., A. Bezos, L. Dosso, J. Escartin, M. Cannat, and C. Mevel (2013), Atypically depleted upper mantle component revealed by Hf isotopes at Lucky Strike segment, Chem. Geol., 341, 128-139.
-
Harvey, J., A. Gannoun, K. W. Burton, N. W. Rogers, O. Alard, and I. J. Parkinson (2006), Ancient melt extraction from the oceanic upper mantle revealed by Re–Os isotopes in abyssal peridotites from the Mid-Atlantic ridge, Earth Planet. Sci. Lett., 244, 606-621.
-
Huismans, R., and C. Beaumont (2011), Depth-dependent extension, two-stage breakup and cratonic underplating at rifted margins, Nature, 473, 74-78.
-
Huismans, R. S., and C. Beaumont (2014), Rifted continental margins: The case for depth-dependent extension, Earth Planet. Sci. Lett., 407, 148-162.
-
Ishikawa, A., D. G. Pearson, and C. W. Dale (2011), Ancient Os isotope signatures from the Ontong Java Plateau lithosphere: Tracing lithospheric accretion history, Earth Planet. Sci. Lett., 301, 159-170.
-
Kaban, M. K., W. D. Mooney, and A. G. Petrunin (2015), Cratonic root beneath North America shifted by basal drag from the convecting mantle, Nat. Geosci., 8, 797-800.
-
King, S. D., and J. Ritsema (2000), African hot spot volcanism: small-scale convection in the upper mantle beneath cratons, Science, 290, 1137-1140.
-
Lenardic, A., L. Moresi, and H. Mühlhaus (2000), The role of mobile belts for the longevity of deep cratonic lithosphere: the crumple zone model, Geophys. Res. Lett., 27, 1235-1238.
-
Liao, J., T. Gerya, and Q. Wang (2013), Layered structure of the lithospheric mantle changes dynamics of craton extension, Geophys. Res. Lett., 40, 5861-5866.
-
Liao, J., and T. Gerya (2014), Influence of lithospheric mantle stratification on craton extension: Insight from two-dimensional thermo-mechanical modeling, Tectonophysics, 631, 50-64.
-
Liu, C. Z., J. E. Snow, E. Hellebrand, G. Brügmann, A. V. D. Handt, A. Büchl, and A. W. Hofmann (2008), Ancient, highly heterogeneous mantle beneath Gakkel ridge, Arctic Ocean, Nature, 452, 311-316.
-
Müller, R. D., M. Sdrolias, C. Gaina, and W. R. Roest (2008), Age, spreading rates, and spreading asymmetry of the world's ocean crust, Geochem. Geophys. Geosyst., 9, Q4006, 10.1029/2007GC001743
-
O'Reilly, S. Y., M. Zhang, W. L. Griffin, G. Begg, and J. Hronsky (2009), Ultradeep continental roots and their oceanic remnants: A solution to the geochemical “mantle reservoir” problem?, Lithos, 112, 1043-1054.
-
Poudjom Djomani, Y. H., S. Y. O Reilly, W. L. Griffin, and P. Morgan (2001), The density structure of subcontinental lithosphere through time, Earth Planet. Sci. Lett., 184, 605-621.
-
Selway, K., H. Ford, and P. Kelemen (2015), The seismic mid-lithosphere discontinuity, Earth Planet. Sci. Lett., 414, 45-57.
-
Selway, K. (2015), Negligible effect of hydrogen content on plate strength in East Africa, Nat. Geosci., 8, 543-546.
-
Sodoudi, F., X. Yuan, R. Kind, S. Lebedev, J. M. C. Adam, E. Kästle, and F. Tilmann (2013), Seismic evidence for stratification in composition and anisotropic fabric within the thick lithosphere of Kalahari Craton, Geochem. Geophys. Geosyst., 14, 5393-5412.
-
Tharimena, S., C. A. Rychert, and N. Harmon (2016), Seismic imaging of a mid-lithospheric discontinuity beneath Ontong Java Plateau, Earth Planet. Sci. Lett., 450, 62-70.
-
Thybo, H., and E. Perchuć (1997), The seismic 8° discontinuity and partial melting in continental mantle, Science, 275, 1626-1629.
-
Wang, Z., Kusky, T., and F. Capitanio (2017), Ancient continental lithosphere dislocated beneath ocean basins along the mid-lithosphere discontinuity: a hypothesis. Geophys. Res. Lett., 44, 9253-9260.
-
Wölbern, I., G. Rümpker, K. Link, and F. Sodoudi (2012), Melt infiltration of the lower lithosphere beneath the Tanzania craton and the Albertine rift inferred from S receiver functions, Geochem. Geophys. Geosyst., 13, 1-20.
-
Yuan, H., and B. Romanowicz (2010), Lithospheric layering in the North American craton, Nature, 466, 1063-1068.
last updated 24th July, 2018 |