 |
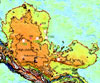 |
Plume
vs. Alternative Hypotheses for the Origin
of the Ontong Java Plateau
|
Modified from Tejada,
M.L.G., Mahoney, J.J., Castillo, P.R., Ingle,
S., Sheth, H.C. and Weis, D., 2004. Pin-Pricking
the Elephant: evidence on the origin of the
Ontong Java Plateau from Pb-Sr-Hf-Nd isotopic
characteristics of ODP Leg 192 basalts. In:
J.G. Fitton, J.J. Mahoney, P.J. Wallace and
A.D. Saunders (Eds.), Origin and evolution
of the Ontong Java Plateau, Geological
Society of London, London, pp. 133-150.
|
Maria
Luisa G. Tejada1
& John
J. Mahoney2
1National
Institute of Geological Sciences, University of the
Philippines, Diliman, Quezon City, 1101 Philippines
2School
of Ocean and Earth Science and Technology, University
of Hawaii at Manoa, Honolulu, Hawai, 96822, USA
mtejada@nigs.upd.edu.ph
& jmahoney@hawaii.edu
|
The Ontong Java Plateau
(OJP), with an area of 2 x 106 km2
and a maximum crustal thickness of more than 30 km
(e.g. Gladczenko
et al.,
1997; Richardson
et al.,
2000; Miura et al., 2004; J.G. Fitton
& M.F. Coffin, pers. comm. 2003), is
the largest of several massive oceanic plateaus that
appeared at equatorial-to-mid-southern latitudes in
the Pacific Basin between the latest Jurassic and
Late Cretaceous. Of these, the OJP is by far the best
sampled by drilling (Figure 1). Four ODP Leg 192 sites
on the main or high plateau penetrated 65-217 m of
basaltic basement, and three earlier DSDP and ODP
sites penetrated 9-149 m. In comparison, only 1-2
basement sites each, with penetrations of 0.9-46 m,
have been drilled on the Manihiki Plateau, Magellan
Rise, Hess Rise, and Shatsky Rise. In addition to
the OJP drill sites, plateau basement sections as
thick as 3.5 km have been sampled in the islands of
Malaita, Santa Isabel, and San Cristobal in the Solomon
Islands along the southern margin of the OJP.
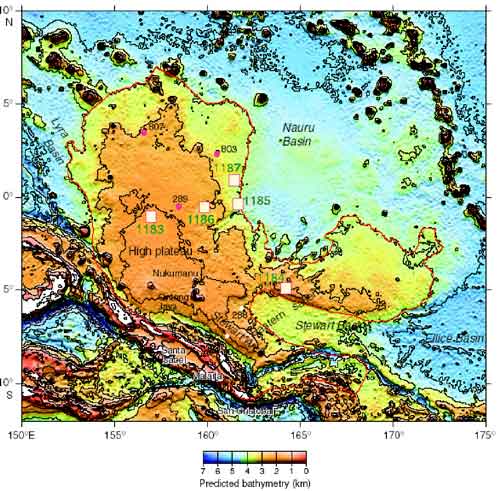
Figure 1: Basement
drill sites on the OJP. Squares represent ODP drill
sites 1183, 1186, 1185 and 1187 and pink dots represent
previous DSDP and ODP drill sites. Click on figure
for higher-resolution enlargement.
The origin of the OJP
and the other Pacific plateaus is debated. Among the
mechanisms proposed are:
-
cataclysmic melting
in the inflated heads of newly risen mantle plumes
(e.g., Richards et al., 1989) or even a
single “super” plume (Larson,
1991),
-
formation above
near-ridge plume tails over much longer periods
of time ( e.g., Mahoney & Spencer, 1991;
Ito
& Clift,
1998),
-
plate separation
above extensive, near-solidus, but non-plume regions
of shallow asthenosphere (e.g. Anderson et al.,
1992; Smith & Lewis, 1999; Hamilton,
2002), and
-
asteroid or comet
impact ( Rogers,
1982).
The variety of models
that has been applied in part reflects a lack of detailed
knowledge of Pacific plateau composition and age. This
in turn is a result of the very sparse sampling of crustal
basement. |
The OJP lavas are low-K tholeiitic
basalts with only a small range of major element,
trace element and Nd-Pb-Sr-Hf isotopic composition
(Figure 2), a rather surprising result in view of
the immensity of the plateau (e.g., Mahoney et
al., 1993; Tejada et al., 1996, 2002,
2004; Babbs, 1997; Neal
et al.,
1997; Fitton & Godard, 2004). All
of these basalts are distinct from both OIB (ocean
island basalt) and Pacific N-MORB (normal mid-ocean-ridge
basalt). They have low, N-MORB-like concentrations
of many incompatible elements but OIB-like isotopic
signatures comparable to those of the Hawaiian shield
volcanoes of Kilauea and Mauna Loa; moreover, unlike
either N-MORB or OIB, their incompatible-element patterns
(normalized to estimated primitive mantle) and rare-earth
patterns are relatively flat (Figure 3). Dating by
40Ar-39Ar suggests most of the
OJP was emplaced in a short period around 120 Ma (Mahoney
et al., 1993; Tejada et al., 1996, 2002;
Parkinson et al., 2001; Chambers et al.,
2004).
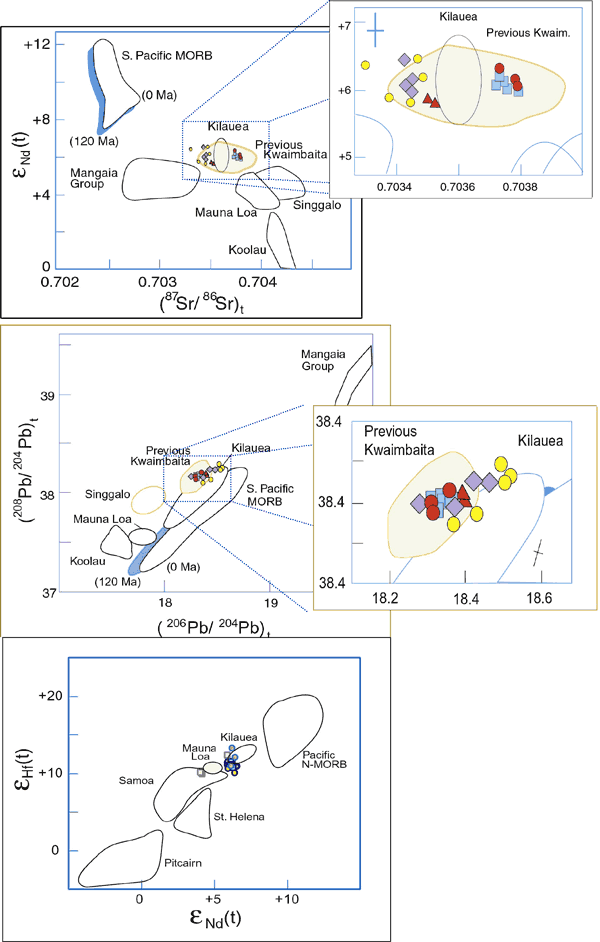
Figure 2: Sr-Nd-Pb-Hf
isotope data for the OJP showing the two isotopic
groups of lavas (orange outline) and the new data
from Leg 192: Site 1183 (yellow circles); Site 1186
(diamond); Site 1185 (red circles) and Site 1187 (blue
squares).
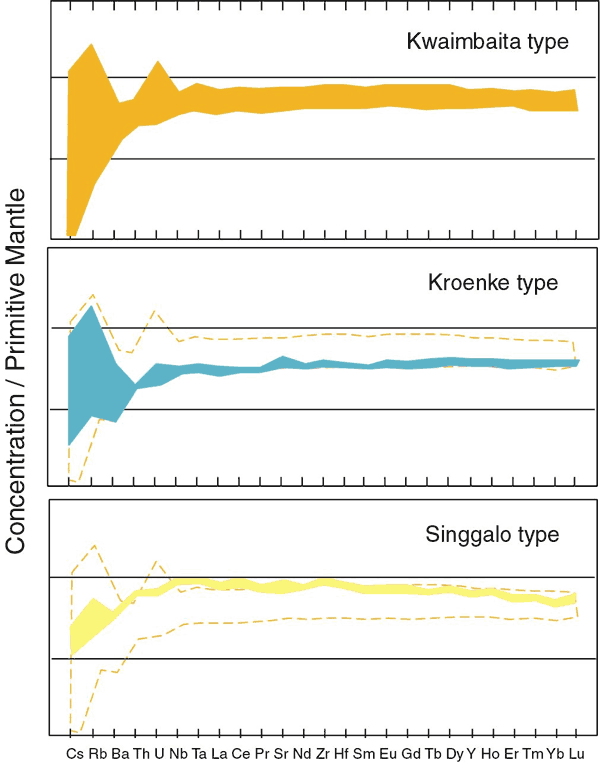
Figure 3: Primitive-mantle-normalized-incompatible-element
pattern for OJP lavas. Normalising values are taken
from Sun & McDonough (1989). The patterns for
Kwaimbaita-type basalt are reproduced, as orange outlines,
on all the other diagrams for comparison. Note the
similarity in the shape of the patterns for Kwaimbaita-
and Kroenke-type basalt and the slight relative enrichment
in the more incompatible elements shown by the Singgalo-type
basalts (Site 807, unit A). Modified from Fitton &
Goddard (2004).
Despite the limited
compositional variability, two isotopically distinct,
stratigraphically separate groups of basalt, termed
the Kwaimbaita and Singgalo types (Tejada
et al.,
2002), have been identified at widely separated
locations. A third, rather primitive magma type termed
the Kroenke-type was found at ODP Sites 1185 and 1187;
isotopically, Kroenke-type basalts are indistinguishable
from the Kwaimbaita type and are likely to be parental
to the Kwaimbaita. Both Singgalo and Kwaimbaita/Kroenke
magma types appear to be the products of high fractions
of partial melting. The most recent estimates of the
average amount of partial melting for the Kwaimbaita
and Kroenke types are between 25 and 31% (Fitton
& Godard, 2004; Herzberg, 2004),
whereas the Singgalo type appears to represent slightly
lesser amounts (Mahoney et al., 1993; Neal
et al.,
1997; Tejada, 1998). On the basis of
present sampling, it appears that Kwaimbaita-type
magmas dominate the upper portion of the plateau’s
crust. Furthermore, Kwaimbaita-type lavas fill the
large Nauru and East Mariana basins adjacent to the
OJP on the north and east (e.g., Mahoney,
1987; Castillo et al., 1991, 1994). The small
isotopic range defined by lavas from the OJP and surrounding
basins indicates an enormous, isotopically homogeneous
(relative to the scale of melting) mantle source (Tejada
et al., 1996, 2002;
Neal
et al.,
1997).
|
What might be the explanation
for the particular combination of isotopic and incompatible-element
characteristics in the mantle source of the OJP?
The flat primitive-mantle-normalized
patterns defined by alteration-resistant incompatible
elements in the Kwaimbaita- and Kroenke-type basalts
(see Fitton & Godard, 2004) point to
a mantle source not too different from estimated primitive
mantle in most of its inter-element ratios. However,
the observed isotopic values (e.g., eNd(t)
~ +6) are clearly far-removed from those estimated
for primitive mantle (eNd = 0). For some oceanic plateaus,
the general explanation given is in terms of variable
mixing of OIB mantle end-member types, with or without
involvement of MORB-type mantle (e.g., Kerr
et al.,
2002). However, no evidence for involvement of
MORB-type mantle has yet been found in the OJP. Although
mixing involving, for example, an EM-1-like end-member
and anciently recycled oceanic lithosphere can explain
the observed OJP isotopic ratios, there are no discernible
mixing arrays in the data, which would be expected
for this model, and no support is provided by trace
or major element modeling (Tejada
et al.,
2002).
Could the Kwaimbaita/Kroenke source
represent originally primitive mantle (i.e.,
originally of bulk silicate Earth composition) that
underwent minor fractionation long ago and evolved
isotopically in an essentially closed-system manner
until the early Aptian? A simple, two-stage evolution
model that assumes a mantle fractionation event in
the 3-4 Ga range can reproduce the Sr, Pb, Hf, and
Nd isotopic characteristics of the Kwaimbaita- and
Kroenke-type basalts (Figure 4). In this model, the
first stage of isotopic evolution occurs in a reservoir
of primitive-mantle chemical and isotopic composition
until about 3 Ga, when a fractionation event, perhaps
the removal of a small (~ 1%) partial melt fraction
occurs, modifying the reservoir’s chemical composition
slightly. Subsequent closed-system isotopic evolution
transpires in this slightly modified mantle reservoir
until the eruption of the OJP lavas at 120 Ma (for
details of this model, see Tejada et al.,
2004). Trace element modeling is consistent with this
model (Fitton & Godard, 2004).
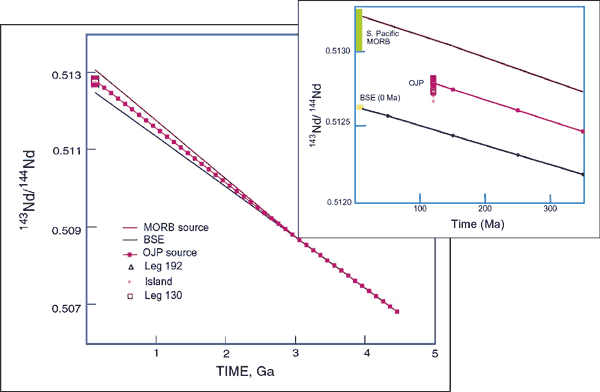
Figure 4: Two-stage
modeling results for the OJP. The first stage of isotopic
evolution occurs until about 3 Ga when 1% partial
melting modified its composition slightly. The second
stage is a closed-system evolution of this modified
mantle until it melted at 120 Ma ago to form the OJP.
|
Arguably
the most likely part of the planet in which a large
volume of ancient, chemically near-primitive material
might survive would be the lower mantle. Recent seismological
results suggest the bottom ~1000 km of today’s
mantle may be chemically distinct, separated from the
rest of the mantle by a thermochemical interface with
large undulations, and that most of the time little
mixing occurs across the interface (e.g., Kellogg
et al., 1999). Hypotheses involving an ultimately
lower-mantle origin for the OJP, of course, inevitably
are coupled to plume-head or mantle-overturn models.
The isotopic homogeneity of the Kwaimbaita- and Kroenke-type
lavas is consistent with recent models of plume formation
in the lower mantle that suggest large plume heads should
be well mixed, should entrain little non-plume mantle
during ascent, and thus should be significantly more
homogeneous than plume tails (e.g., Van Keken,
1997; Farnetani et al., 2002). The OIB-like
isotopic signature and the apparently rapid formation
of the bulk of the OJP around 120 Ma by high-degree
partial melting also are consistent with predictions
of plume-head models (e.g., Richards et al.,
1989; Campbell & Griffiths, 1990; Campbell,
1998).
The problem
with the plume hypothesis for the OJP is that……
However, several significant
discrepancies exist between observation and model, including
the following (Tejada
et al.,
2002):
- the recurrence of compositionally
Kwaimbaita-like tholeiitic basalts at ~90 Ma, after
an apparent eruptive hiatus of ~30 m.y.;
- the lack of a post-plateau seamount
chain corresponding to the plume-tail stage of hotspot
development theorized to follow the plume-head stage;
- the lack of any presently active
hotspot that can be linked unambiguously to the
plateau;
- that post-eruptive subsidence of
the plateau appears to have been much less than
expected for oceanic lithosphere; and
- the substantial water depths at which
most OJP lavas appear to have been erupted (below
the calcite compensation depth in some cases) are
contrary to predictions of shallow or subaerial
emplacement. The calcite compensation depth is the
depth in the ocean where the rate of calcium carbonate
dissolution exceeds the rate of deposition. This
means that if the oceanic floor or seamount top
is above the calcite compensation depth, limestone
layers may be deposited on it, but if they are below,
limestone does not accumulate. In the present Pacific
ocean, this level is at 4,000-5,000 meters.
Many of these discrepancies
may provisionally be accommodated by assuming a number
of case-specific modifications to the standard plume-head
model, but the sheer number of ad hoc modifications
needed indicates to us that the model may not be appropriate
at all for the OJP. |
Can plate
tectonic processes and perisphere explain the origin
of the OJP?
Non-plume enthusiasts
have suggested mechanisms for the origin of large igneous
provinces that include focusing of melt flow, unusually
fertile mantle (see also PT
Processes page), large-scale melt ponding, “EDGE”
and rift-related processes (see also EDGE
page), and a partially molten shallow mantle (e.g.,
Anderson, 1998). The plate-separation hypothesis
(e.g., Anderson et al., 1992; Smith &
Lewis, 1999) posits an extensive layer of shallow,
volatile-rich, near-solidus, OIB-like (but not plume
derived) asthenosphere (“perisphere”) to
have resided beneath a region of the Pacific lithosphere
that was rifted suddenly by a ridge jump around 120
Ma, causing cataclysmic melting. However, the pre-120
Ma seafloor within several hundred kilometers of the
OJP is not much older than the plateau itself, having
been formed only ~ 2 – 35 m.y. earlier (e.g.,
Taylor, 1978; Nakanishi
et al.,
1992). Thus, during this period, a spreading system
was not too distant from the (future) location of the
OJP. It is difficult to understand why such perisphere,
assuming it existed, was not drained earlier by the
nearby ridge. Also, the hypothesis predicts that normal
MORB-type mantle lying just beneath the perisphere should
have been tapped progressively more as OJP volcanism
proceeded; yet, as noted above, no evidence of MORB-type
mantle has been found thus far in OJP basalts. Indeed,
the Singgalo-type basalts that compose the topmost part
of the lava pile in several widespread locations are
even less MORB-like than the Kwaimbaita and Kroenke
types. Furthermore, OJP lavas are notably poor, not
rich, in volatiles (Roberge
et al.,
2004). |
Why not
meteorite impact instead of plume impact?
Well before either the
plume-head or plate-separation hypotheses were proposed,
Rogers
(1982) suggested that the Pacific plateaus represent
massive outpourings of basalt formed by the cataclysmic
excavation of asthenosphere by large but rare meteorite
impacts. We find this hypothesis attractive in that
it can explain the absence of a post-plateau seamount
chain and any obvious present-day hotspot that can be
linked with the OJP. Also, the apparent lack of large
areas at shallow water depths during the construction
of the OJP is not necessarily a problem, because inherently
hotter than normal mantle is not required for widespread
magmatism. Nor are huge amounts of volatile-rich (or
eclogitic) mantle necessary. Moreover, the limited range
of elemental and isotopic variation in both the Singgalo-
and Kwaimbaita-type basalts might be attributable in
part to melt homogenization in extensive magma pools
created by the impact. Another potential advantage of
the impact hypothesis is that it may help explain the
enigmatic 300-km-thick, seismically slow mantle “root”
beneath the OJP (Ingle
& Coffin,
2004).
However, just as with
the plate separation hypothesis, this hypothesis does
not explain why OJP lavas lack any resemblance to Pacific
MORB of the same age. Large-volume, high-degree partial
melting of the upper few hundred kilometers of sub-seafloor
mantle resulting from a large impact would ordinarily
be expected to produce basalt with essentially N-MORB-type
isotopic and incompatible element characteristics. Although
sampled in relatively few places, pre-OJP Pacific MORB
are isotopically and chemically indistinguishable from
modern Pacific MORB (Janney & Castillo,
1997; Mahoney et al., 1998). In contrast, the
enormous volumes of OJP basalt have OIB-like isotopic
signatures and rather flat incompatible-element patterns,
and there is as yet no evidence for any involvement
of MORB-type mantle in the plateau (see above). Note
also that neither chondritic, achondritic, or iron meteorites
have a suitable combination of Sr-Pb-Nd isotopic characteristics
(e.g., Kerridge & Matthews, 1988, and references
therein) to explain the isotopic signature of the OJP
basalts via contamination of MORB-type mantle with meteoritic
material, even assuming a significant amount of such
material remained at the impact site.
To reconcile the geochemical
evidence with the impact hypothesis, the impact site
can be assumed to have fortuitously been located above
a geochemically anomalous region of asthenosphere containing
a large amount of OIB-type mantle (cf. Ingle
& Coffin,
2004). However, suitable isotopic compositions are
notably rare among modern hotspots and non-hotspot volcanic
areas in the South Pacific, where plate reconstructions
put the original location of the OJP. It can be speculated
further that a large impact triggered a deep mantle
plume beneath the impact site (e.g., Alt et al.,
1988; Glikson,
1999), although it is not clear whether significant
initial uplift of the plateau's surface would result
or not.
An additional challenge
for the impact hypothesis is the reportedly systematic
patterns in the gravity field and bathymetry of the
OJP that Winterer & Nakanishi (1995), Neal
et al.
(1997), and Kroenke et al. (2004) have
suggested represent a seafloor spreading fabric. This
interpretation remains to be evaluated rigorously but,
if correct, is very difficult to reconcile with the
expected widespread destruction and disruption of preexisting
oceanic lithosphere by a large impact, or with the short-lived
outpouring of magma following an impact, which would
be too rapid to allow formation of significant amounts
of new seafloor.
To our knowledge, key
features diagnostic of other large impact events, such
as microspherules and siderophile element anomalies,
have not been found in terrestrial or marine sediments
of OJP age. Moreover, in contrast to the impact-linked
Cretaceous-Tertiary boundary, no mass extinction occurred
at the time of OJP formation. Further, it is not clear
that a sufficiently large object has been available
in Earth’s vicinity in the last few hundred million
years. The Cretaceous-Tertiary boundary impact created
the largest known Phanerozoic impact crater, the ~ 200
km-wide crater at Chicxulub, Mexico, which is thought
to have been formed by an object ~ 10 km in diameter
(Grieve & Therriault, 2000, and references
therein). Any object that could trigger the formation
of the huge OJP must have been considerably larger.
However, no near-Earth objects larger than 40 km in
diameter are observed today, and only two are larger
than 20 km (Binzel et al., 2002). Venus, with
a surface age estimated at ~ 600 Ma (e.g., Nimmo
& McKenzie, 1998), lacks any impact craters
larger than Chicxulub (Schaber et al., 1992),
whereas all lunar craters larger than ~ 100 km in diameter
appear to be older than ~ 800 Ma (e.g., Eberhardt
et al., 1973; Grier et al., 2001). Nevertheless,
despite these potentially serious problems with the
impact hypothesis, we regard it as deserving of further
study in view of the difficulties encountered with any
simple form of the plume-head or other proposed models
for the OJP. |
Back to
square one…
Presently, it appears
that no single model for the origin of the OJP can explain
all of the geochemical, geophysical, and geodynamical
observations. This apparent failure makes the OJP one
of the most challenging and potentially fruitful sites
for further scientific investigation in the near future. |
-
Alt, D., Sears,
J. W. & Hyndman, D. W., 1988. Terrestrial maria:
the origins of large basalt plateaus, hotspot tracks,
and spreading ridges. Journal of Geology,
96, 647- 662.
-
Anderson,
D.L., The EDGES of the mantle, in: M. Gurnis, M.E.
Wysession, E. Knittle, B.A. Buffet (Eds.), The
Core-Mantle Boundary Region, Geodynamics 28,
AGU, Washington, D.C, 255-271, 1998.
-
Anderson,
D. L., Zhang, Y.-S. & Tanimoto, T., 1992. Plume
heads, continental lithosphere, flood basalts and
tomography. In: Storey, B.C., Alabaster, T., and
Pankhurst, R.J. (eds.) Magmatism and the Causes
of Continental Break-up, Geological Society,
London, Special Publications, 68, 99-124.
-
Babbs, T.
L., 1997. Geochemical and petrological investigations
of the deeper portions of the Ontong Java Plateau:
Malaita, Solomon Islands. Ph.D. thesis, University
of Leicester.
-
Binzel, R.
P., Lupishko, D. F., Di Martino, M., Whiteley, R.
J. & Hahn, G. J., 2002. Physical properties
of near-Earth objects. In: Bottke, W., Cellino,
A., Paolicchoi, P., and Binzel, R. P. (eds.) Asteroids
III, University of Arizona Press, Tucson, pp.
255-271.
-
Campbell,
1998. The mantle’s chemical structure: Insights
from the melting products of mantle plumes. In Jackson,
I. (ed.) The Earth’s Mantle: Composition,
Structure and Evolution, 566 p.
-
Campbell,
I. H. & Griffiths, R. W., 1990. Implications
of mantle plume structure for the evolution of flood
basalts. Earth and Planetary Science Letters,
99, 79-93.
-
Castillo,
P. R., Pringle, M. S. & Carlson, R. W., 1994.
East Mariana Basin tholeiites: Jurassic ocean crust
or Cretaceous rift basalts related to the Ontong
Java plume? Earth and Planetary Science Letters,
123, 139-154.
-
Chambers,
L. M., Pringle M. S. & Fitton J. G. 2004. Phreatomagmatic
eruptions on the Ontong Java Plateau: an Aptian
40Ar/39Ar age for volcaniclastic
rocks at ODP Site 1184. In Fitton, G., Mahoney,
J., Wallace, P. & Saunders, A. (eds.), Origin
and Evolution of the Ontong Java Plateau, Geological
Society, London, Special Publications, in press.
-
Eberhardt,
P., Stetler, A., Geiss, J., Grosler & N., 1973.
How old is the lunar crater Copernicus?, Moon,
8, 1-4-114.
-
Farnetani,
C. G., Legras, B. & Tackley, P. J., 2002. Mixing
and deformations in mantle plumes. Earth and
Planetary Science Letters, 196,
1-15.
-
Fitton, J.
G. & Godard, M., 2004. Origin and evolution
of magmas on the Ontong Java Plateau. In Fitton,
G., Mahoney, J., Wallace, P. & Saunders, A.
(eds.), Origin and Evolution of the Ontong Java
Plateau, Geological Society, London, Special
Publications, in press.
-
-
-
Grier, J.
A., McEwan, A. S., Lucey, P. G., Milazzo, M., and
Strom, R. G., 2001. Optical maturity of ejecta from
large rayed lunar craters. Journal of Geophysical
Research, 106, E12: 32,847-32,862.
-
Grieve, R.
& Therriault, A., 2000. Vredefort, Sudbury,
Chicxulub: three of a kind?, Annual Review of
Earth and Planetary Sciences, 28,
305-338.
-
Hamilton,
W. B., 2003. The closed upper-mantle circulation
of plate tectonics. In Stein, S., and Freymueller,
J.T. (eds.) Plate Boundary Zones. Geodynamics
Series Monograph, American Geophysical Union,
30, 359-410.
-
Herzberg,
C., 2004. Partial melting below the Ontong Java
Plateau. In Fitton, G., Mahoney, J., Wallace, P.
& Saunders, A. (eds.), Origin and Evolution
of the Ontong Java Plateau, Geological Society,
London, Special Publications, in press.
- Ingle,
S. and Coffin, M.F., 2004. Impact origin for the greater
Ontong Java Plateau? Earth and Planetary Science
Letters, 218: 123-134.
-
-
Janney, P.
E. & Castillo, P. R., 1997. Geochemistry of
Mesozoic Pacific MORB: constraints on melt generation
and the evolution of the Pacific upper mantle. Journal
of Geophysical Research, 102,
5207-5229.
-
Jones, A.
P., Price, G. D., Price, N. J., DeCarli, P. S. &
Clegg, R. A., 2002. Impact induced melting and the
development of large igneous provinces. Earth
and Planetary Science Letters, 202,
551-561.
-
Kellogg, L.
H., Hager, B. H. & van der Hilst, R. D., 1999.
Compositional stratification in the deep mantle.
Science, 283, 1881-1884.
-
Kerr,
A. C., Tarney, J., Kempton, P. D., Spadea, P., Nivia,
A., Marriner, G. F. & Duncan, R. A., 2002. Pervasive
mantle plume head heterogeneity: evidence from the
Late Cretaceous Caribbean-Colombian oceanic plateau.
Journal of Geophysical Research, 107,
10,1029-10,1041.
-
Kerridge,
J. F. & Matthews, M. S. (eds.), 1988. Meteorites
and the Early Solar System, University of Arizona
Press, Tucson, 1269 pp.
-
Kroenke, L.
W., Wessel, P. & Sterling, A., 2004. Motion
of the Ontong Java Plateau in the hotspot frame
of reference: 120 Ma to the present. In Fitton,
G., Mahoney, J., Wallace, P. & Saunders, A.
(eds.), Origin and Evolution of the Ontong Java
Plateau, Geological Society, London, Special
Publications, in press.
-
Larson, R.
L., 1991. Latest pulse of the earth: evidence for
a mid-Cretaceous superplume. Geology, 19,
547-550.
-
Mahoney, J.
J., 1987. An isotopic survey of Pacific oceanic
plateaus: implications for their nature and origin.
In: Keating, B., Fryer, P., Batiza, R. & Boehlert,
G. (eds.) Seamounts, Islands, and Atolls.
Geophysical Monograph, American Geophysical Union,
43, 207-220.
-
Mahoney, J.
J. & Spencer, K. J., 1991. Isotopic evidence
for the origin of the Manihiki and Ontong Java oceanic
plateaus. Earth and Planetary Science Letters,
104, 196-210.
-
Mahoney, J.
J., Storey, M., Duncan, R. A., Spencer, K. J. &
Pringle, M., 1993. Geochemistry and geochronology
of the Ontong Java Plateau. In: Pringle, M., Sager,
W., Sliter, W., and Stein, S. (eds.) The Mesozoic
Pacific. Geology, Tectonics, and Volcanism.
Geophysical Monograph, American Geophysical Union,
77, 233-261.
-
Mahoney, J.
J., Frei, R., Tejada, M. L. G., Mo, X. X., Leat,
P. T. & Nägler, T. F., 1998. Tracing the
Indian Ocean mantle domain through time: isotopic
results from old West Indian, East Tethyan, and
South Pacific seafloor. Journal of Petrology,
39, 1285-1306.
-
Miura, S.,
Suyehiro, K., Shinohara, M., Takahashi, N., Araki,
E. & Taira, A., 2004. Seismological structure
and implications of double convergence and oceanic
plateau collision of Ontong Java Plateau and Solomon
Island arc from ocean bottom seismometer-airgun
data. Tectonophysics, in press.
-
-
Neal,
C. R., Mahoney, J. J., Kroenke, L. W., Duncan, R.
A. & Petterson, M. G., 1997. The Ontong Java
Plateau. In: Mahoney, J. J. and Coffin, M. F. (eds.)
Large Igneous Provinces: Continental, Oceanic,
and Planetary Flood Volcanism. Geophysical
Monograph, American Geophysical Union, 100,
183-216.
-
Nimmo, F.
& McKenzie, D., 1998. Volcanism and tectonics
on Venus. Annual Review of Earth and Planetary
Sciences, 26, 23-51.
-
Richards,
M. A., Duncan, R. A. & Courtillot, V., 1989.
Flood basalts and hot-spot tracks: plume heads and
tails. Science, 246, 103-107.
-
-
Roberge,
J., White, R. V. and Wallace, P. J. , Volatiles
in submarine basaltic glasses from the Ontong Java
Plateau (ODP Leg 192): implications for magmatic
processes and source region compositions, in: Origin
and Evolution of the Ontong Java Plateau, Fitton
J.G., Mahoney J.J., Wallace P.J. and Saunders
A.D., Eds., Geological Society (London) Special
Publication, 229, 239-257, 2004.
-
-
Saunders,
A. D., Storey, M., Kent, R. W. & Norry, M. J.,
1992. Consequences of plumelithosphere interactions.
In Storey, B. C., Alabaster, T., and Pankhurst,
R. J. (eds.) Magmatism and the Causes of Continental
Break-Up, Geological Society, London, Special
Publications, 68, 41-59.
-
Schaber, G.
G., Strom, R. G., Moore, H. J., Soderblom, L. A.,
Kirk, R. L., et al., 1992. Geology and distribution
of impact craters on Venus: what are they telling
us? Journal of Geophysical Research, 97,
E8: 13,257-13,301.
-
Smith, A.
D. & Lewis, C., 1999. The planet beyond the
plume hypothesis. Earth-Science Reviews,
48, 135-182.
-
Taylor, B.,
1978. Mesozoic magnetic anomalies in the Lyra Basin.
Eos, Transactions of the American Geophysical
Union, 59, 320.
-
Tejada, M.
L. G., 1998. Geochemical studies of Pacific
oceanic plateaus: the Ontong Java Plateau and Shatsky
Rise. Ph.D. thesis, University of Hawaii.
-
Tejada, M.
L. G., Mahoney, J. J., Duncan, R. A. & Hawkins,
M. P., 1996. Age and geochemistry of basement and
alkalic rocks of Malaita and Santa Isabel, Solomon
Islands, southern margin of Ontong Java Plateau.
Journal of Petrology, 37,
361-394.
-
Tejada,
M. L. G., Mahoney, J. J., Neal, C. R., Duncan, R.
A. & Petterson, M. G., 2002. Basement geochemistry
and geochronology of Central Malaita, Solomon Islands,
with implications for the origin and evolution of
the Ontong Java Plateau. Journal of Petrology,
43, 449-484.
-
Tejada,
M.L.G., Mahoney, J.J., Castillo, P.R., Ingle,
S., Sheth, H.C. and Weis, D., 2004. Pin-Pricking
the Elephant: evidence on the origin of the
Ontong Java Plateau from Pb-Sr-Hf-Nd isotopic
characteristics of ODP Leg 192 basalts. In:
J.G. Fitton, J.J. Mahoney, P.J. Wallace and
A.D. Saunders (Eds.), Origin and evolution
of the Ontong Java Plateau, Geological
Society of London, London, pp. 133-150.
-
Van Keken,
P., 1997. Evolution of starting mantle plumes: a
comparison between numerical and laboratory models.
Earth and Planetary Science Letters, 148,
1-11.
-
Winterer,
E. L. & Nakanishi, M., 1995. Evidence for a
plume-augmented, abandoned spreading center on Ontong
Java Plateau. Abstract, Eos, Transactions of
the American Geophysical Union, 76,
F, 617.
last updated 31st December,
2006 |
|
|