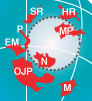 |
Recycling
of the Rodinia supercontinent in the Cretaceous
Ontong Java Plateau? |
Akira
Ishikawa1,2, Takeshi
Kuritani1,3, Akio
Makishima1 & Eizo
Nakamura1
1The
Pheasant Memorial Laboratory for Geochemistry and
Cosmochemistry, Institute for Study of the Earth's
Interior, Okayama University, Tottori, 682-0193,
Japan.
2Arthur
Holmes Isotope Geology Laboratory, Department of
Earth Sciences, Durham University, Durham, DH1 3LE,
UK.
3Graduate
School of Science, Tohoku University, Sendai, 980-8578,
Japan,
akira.ishikawa@durham.ac.uk,
kuritani@mail.tains.tohoku.ac.jp,
max@misasa.okayama-u.ac.jp, eizonak@misasa.okayama-u.ac.jp
Abstract
A rare quartz-garnet clinopyroxenite
xenolith recovered from the island of Malaita in the
southwest Pacific on the Ontong Java Plateau has lower 206Pb/204Pb-143Nd/144Nd
and higher 87Sr/86Sr-207Pb/204Pb
ratios than most oceanic basalts, providing the first
conclusive evidence for an ancient crustal origin for
pyroxenites within the Pacific convective mantle. Constraints
from major and trace-element characteristics, the large
extent of Hf-Nd isotopic decoupling, and the good agreement
of Pb isotopes with the Stacey-Kramers curve, all indicate
that contamination of southern Pacific mantle occurred
by the subduction or delamination of Neoproterozoic
granulite-like lower crust with an age of 0.5-1 Ga.
This crustal recycling could have taken place around
the suture of the Rodinia supercontinent, a part of
which resurfaced during the mantle upwelling responsible
for creating the Cretaceous Ontong Java Plateau.
1. Introduction
The heterogeneity of Earth's mantle,
revealed by the geochemistry of oceanic basalts such
as ocean island basalt (OIB) and mid-ocean ridge basalt
(MORB), has been commonly attributed to recycling of
crustal materials (e.g., Hofmann,
1997; Stracke
et al.,
2003) which are thought to be present as eclogitic/pyroxenitic
bodies within the convecting peridotite-dominated mantle
(Allègre & Turcotte,
1986). During
the last decade, the role of eclogitic/pyroxenitic
sources in the formation of mantle-derived magmas has
received an increasing amount of attention in a number
of detailed investigations (e.g., Hauri,
1996; Hirschmann & Stolper,
1996). However, their nature, origin, recycling
timescales and even their existence remain controversial
because the determination of the magma sources is not
straightforward. Mafic layers in orogenic peridotite
massifs and eclogite xenoliths in kimberlites have
frequently been taken as an analogue for the aging
of old oceanic crust (e.g.,
Jacob
et al.,
2005; Pearson & Nowell,
2004). The isotopic compositions of these rocks
show extremely large variations compared with those
observed in oceanic basalts, reflecting their derivation
from ancient subcontinental lithosphere. This suggests
that their involvement in basalt source regions in
the convecting mantle is questionable and underlines
the importance of natural samples of pyroxenite/eclogite
derived from known oceanic locations for assessing
better the origin of oceanic basalts and heterogeneities
in the mantle.
Here we present a study of a suite
of garnet pyroxenite xenoliths from Malaita, Solomon
Islands, as a convincing example of recycled material
from within the Pacific convective mantle. This argument
is founded on three main lines of evidence:
- The Malaitan xenoliths were brought up to the
surface by a 34-Ma alnöite magma intruded
within the southwestern margin of the 120-Ma Ontong
Java Plateau (e.g., Fitton & Godard,
2004; Tejada et al.,
2004) in an essentially
oceanic setting (Figure 1). This is evidence that
the xenoliths are fragments of oceanic lithosphere
influenced by the plateau emplacement, and not
associated with any known subducting slab or slab-related
structures.
- Thermobarometric
analyses of the suites of garnet pyroxenite xenoliths
reveal derivation from the bottom of the lithosphere
(110-120 km depth; Ishikawa
et al.,
2004) overlain by the pre-existing
Jurassic oceanic lithosphere (ca. 160 Ma; Ishikawa
et al.,
2005). This indicates that isolation
of the garnet pyroxenites from the convective mantle
occurred between 160 and 34 Ma, probably associated
with the 120-Ma plateau emplacement.
- The extent
of petrochemical variation in the garnet pyroxenites
is illustrated by the presence of quartz-garnet
clinopyroxenite, which cannot be regarded as a
high-pressure cumulate or melt from normal peridotitic
mantle. This suggests that the rock originated
from normative quartz-rich basaltic material, most
likely ancient crust stored in the Pacific convective
mantle.
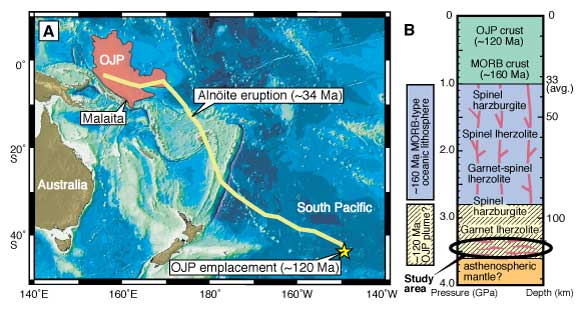
Figure 1: (A) Location
map of Ontong Java Plateau (OJP) and its hypothetical
hot spot trail (Kroenke
et al., 2004). The bathymetric map was reproduced
from the GEBCO Digital Atlas published by the British
Oceanographic Data Centre. (B) Inferred stratigraphic
succession beneath the Ontong Java Plateau based
on a thermobarometric study of Malaitan xenoliths
(Ishikawa
et al., 2004). Click here or on
Figure for enlargement.
2. Results
2.1 Introduction
We analyzed Sr-Nd-Hf-Pb
isotope compositions and trace-element concentrations
of pure clinopyroxene and garnet separates (Pb isotopes
are only for clinopyroxene) from one quartz-garnet
clinopyroxenite sample (QGC) and four bimineralic garnet
clinopyroxenite samples (BGC). The mineral compositions
of these rocks reflect their high-temperature equilibration
(1300-1350°C)
as indicated by an apparent lack of compositional gradient
either in major- or trace-element concentrations coherently
partitioned between garnet and clinopyroxene. The attainment
of mineral equilibrium is strikingly demonstrated by
two-point Sm-Nd and Lu-Hf inter-mineral isochron ages,
displaying good agreement with the 34-Ma host eruption
(Figure 2). These data suggest that isotopic equilibrium
within individual xenoliths was preserved at the time
of host alnöite eruption as ambient conditions
exceeded the blocking temperatures of these isotopic
systems. Thus whole-rock compositions reconstructed
from the mineral data and modal estimates are meaningful
in terms of representing their compositional characteristics.
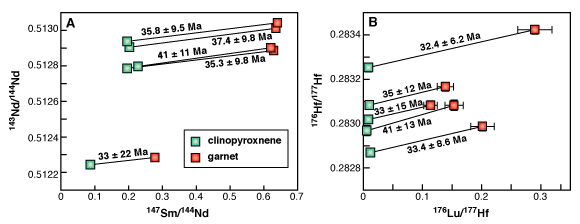
Figure 2: Sm-Nd (A) and Lu-Hf (B)
isotope systematics of Malaita garnet clinopyroxenites.
Lines connect the clinopyroxene and garnet pair from
the same sample.
2.2 Isotopic variability
in garnet clinopyroxenite
In contrast to homogeneous features
on the scale of individual xenolith specimens, the
reconstructed whole-rock data display significant variations
as shown in Sr-Nd-Hf-Pb diagrams (Figure 3). It is
clear that the QGC has a distinctive composition relative
to the BGC. While the Sr-Nd-Hf-Pb isotopic variations
of the BGC are within the MORB-OIB array, the QGC is
characterized by remarkably lower 206Pb/204Pb-143Nd/144Nd
and higher 87Sr/86Sr-207Pb/204Pb
ratios, showing some affinities with EM-1 or DUPAL-type
isotopic signatures (e.g., Hofmann,
1997; Stracke
et al.,
2003). The most striking feature of the QGC can
be seen in an εHf-εNd plot,
displaying great deviation above and to the left of
the terrestrial array defined by the Hf-Nd isotopic
values of a wide variety of crust-mantle samples
(Vervoort
et al.,
1999).
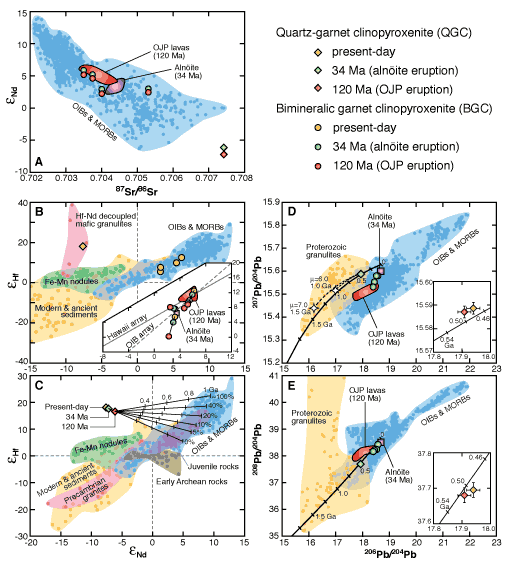
Figure 3: The calculated whole-rock
Sr-Nd (A), Hf-Nd (B, present-day εNd-εHf (C),
initial εNd-εHf) and
Pb (D,
207Pb/204Pb-206Pb/204Pb;
E, 208Pb/204Pb-206Pb/204Pb)
isotope compositions of BGC (circles) and QGC (diamonds)
compared with 120 Ma Ontong Java Plateau (OJP) lavas
and 34 Ma alnöite. References for the background
plots are given in the original paper. Inset in (B)
shows an enlargement of the evolutionary path for BGC
based on present-day parent-daughter ratios. Bold lines
in (C) represent evolutionary paths of Hf-Nd isotopes
(0-1 Ga) for QGC, which yield different trajectories
before 120 Ma according to fractions of extracted melt
(0-100 %). Bold curves in (D) and (E) represent the
evolution of Stacey-Kramers Pb. Inset in (D) and (E)
shows the enlargement of the data of QGC, which fits
well in ca. 0.5 Ga of Stacey-Kramers Pb. Error bars
(2σ of triplicate analyses) indicate that
the present-day and 120 Ma values are almost identical
due to very low present μ and ω values.
Note that the large uncertainty of clinopyroxene-melt
U, Th and Pb partitioning impedes the assessment of
the Pb isotope evolution before 120 Ma. If the 120-Ma
melt extraction event resulted in preferential removal
of U relative to Pb according to ΔU/ΔPb=0.25-0.3,
maximum primary μ=6-7 make the initial
Pb composition approach the 1- to 1.5-Ga point of the
Stacey-Kramers curve in the appropriate time interval
(open stars with dashed lines). However, μ=6-7
is too high relative to those of lower crustal mafic
granulites with anhydrous mineral assemblages. Click
here or
on image to enlarge.
These isotopic differences between
BGC and QGC can be ascribed to the different extent
of chemical reaction against ambient peridotite in
the context of melting of eclogite/pyroxenite bearing
mantle. The petrochemical features of the QGC suggests
that its precursor was a high-pressure liquidus clinopyroxene
produced by melting of basaltic material after extraction
of siliceous melt, and thus the influence of ambient
peridotite is apparently negligible. The BGC may also
represent basaltic residues, but the rocks apparently
underwent extensive chemical mass exchange with ambient
peridotite through liquid percolation and diffusion
in order to reach chemical equilibrium. It should be
noted that the most depleted BGC records very similar
Sr-Nd-Hf-Pb isotopic compositions to those of 120-Ma
Ontong Java Plateau lavas (Tejada et al.,
2004), suggesting
that the abovementioned reaction process operated in
the framework of the plateau generation. This in turn
implies that the magmatism resulted in melting of eclogite/pyroxenite-bearing
mantle as proposed by several previous workers (Ishikawa
et al.,
2004; Korenaga,
2005; Tejada
et al.,
2002). Thus, the isotopic
characteristics of the BGC are best explained if the
primary enriched signatures of the rocks were largely
obscured by the melt-mediated reaction against ambient
peridotite with depleted isotopic characteristics.
2.3 Origin of quartz-garnet
clinopyroxenite
The isotopic characteristics of QGC reflect a long
time-integrated evolution with high Rb/Sr-Lu/Hf and
low Sm/Nd-U/Pb ratios without recent interaction with
ambient peridotite, inferred from the preservation
of the normative quartz-rich composition. When the
Hf-Nd data are viewed at 120 Ma, they approach the
terrestrial array because of the subchondritic Sm/Nd
and superchondritic Lu/Hf ratios (Figure 3C). This
shows that present-day parent-daughter ratios predominantly
reflect ancient fractionation. Assuming that the 120-Ma
magmatism produced the present whole-rock composition
by accumulation of residual clinopyroxene after extraction
of equilibrated batch melt, the series of isotopic
trajectories earlier than 120 Ma can be approximated
by varying the fractions of extracted melt, whose trace
element concentrations can be assessed by clinopyroxene/melt
partitioning data (Johnson,
1998; Pertermann & Hirschmann,
2002; Watson et al.,
1987). Interestingly,
the data devolve back to the terrestrial array in the
upper right quadrant at approximately 1 Ga regardless
of extracted melt fraction.
Hf-Nd decoupling in OIB magmas
is frequently attributed to the involvement of a pelagic
sediment component (e.g., Blichert-Toft
et al.,
1999; Eisele
et al.,
2002), but similar isotopic evolution
and larger extents of Hf-Nd decoupling among crustal
samples have been recognized also in mafic granulite
xenoliths from the lower crust beneath southern Africa
(Schmitz
et al.,
2004) and northern Queensland,
Australia (Vervoort
et al.,
2000). Despite
the fact that these mafic granulites could be generated
through complex multiple melting episodes, they all
consistently possess normative quartz-rich basaltic
compositions, depletion in HFSE relative to the MREE,
and enrichment of LREE relative to the HREE (Figure
4; Rudnick & Taylor, 1987; Schmitz
et al.,
2004). These characteristics can be matched
with potential precursor compositions of QGC estimated
by adding equilibrated batch melt. This leads to the
suggestion that the QGC protolith formed through a
similar lower-crustal differentiation event at about
1 Ga.
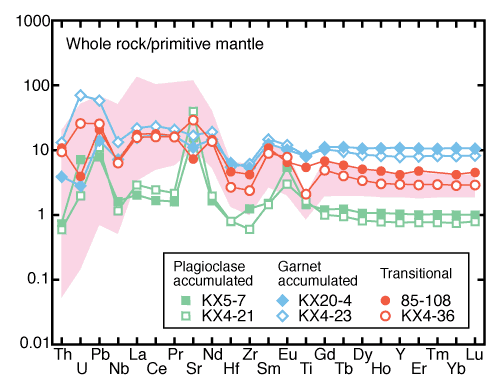
Figure 4: Primitive
mantle-normalized (McDonough & Sun,
1995) trace-element patterns for Hf-Nd decoupled
mafic granulite xenoliths (Rudnick & Taylor, 1987; Schmitz
et al., 2004). Pink field is defined by calculated
whole-rock data of QGC (lower bound) and coexisting
melt compositions estimated by using clinopyroxene/melt
partitioning data (upper bound), which represents the
likely compositional range of the QGC protolith.
Pb isotopic compositions contribute
another important piece of information about the nature
and differentiation age of QGC. In Pb isotope diagrams
(Figure 3D, E), the QGC data plot close to the 0.5-Ga
point on the Stacey-Kramers Pb evolution curve, which
has been regarded as an evolutionary trace for crustal
Pb (Stacey & Kramers,
1975). The most straightforward explanation is
that the protolith lost U and Th and/or gained crustal
Pb at about 0.5 Ga, and subsequent isotopic evolution
was hampered by very low μ (238U/204Pb)
and ω (232Th/204Pb)
values. This is analogous to the typical Pb isotopic
evolution history of Proterozoic mafic granulites,
where their Pb isotope compositions may reflect the
age of the last tectonothermal or orogenic event that
affected the area (e.g., Rudnick & Goldstein,
1990). If this hypothesis is valid, the timing
of removal of the lower crust into the underlying mantle
can be bound by the minimum isolation age of 0.5 Ga
because the subduction of crustal material is an inherent
process in active orogenic zones. Even if lower crustal
delamination facilitated by gravitational instability
is considered, the situation is unchanged because the
process is thought to be restricted to the high-geothermal-gradient
region accompanied by volcanic activity (e.g., Jull & Kelemen,
2001), which may rejuvenate the Pb isotope composition
of delaminating lower crust. From these considerations,
we envisage that protolith formation and subsequent
subduction or delamination of the QGC occurred during
the Neoproterozoic era, at 0.5-1 Ga.
3. Discussion
3.1 Recycling history
of the garnet clinopyroxenite
The recycling timescale and material
documented by the chemical and isotopic compositions
of the QGC are consistent with the presence of Neoproterozoic
lower-crust-like material within the Cretaceous Pacific
mantle. This raises the question of how such material
may have been recycled in the context of the geodynamic
history of the Pacific region. Based on available geologic
records and paleomagnetic data, it has been suggested
that a supercontinent Rodinia formed at ca. 1.1 Ga
and broke apart at about 0.7-0.8 Ga creating the Proto-Pacific
Ocean (Figure 5A; Hoffman,
1991; Weil
et al.,
1998). Despite considerable uncertainty
about the paleogeographic position and configuration
of Rodinia (e.g., Meert & Torsvik,
2003),
most of the 1.0 to 1.1-Ga orogenic belts are proposed
to mark the sutures between various elements of the
supercontinent. Thus, one possible scenario is that
Rodinia supercontinent breakup induced detachment and
dispersal of the overthickened suture zone between
accreted fragments of itself. This is analogous to
recent models suggesting that the breakup of Gondwana
played a major role in the contamination of Atlantic-Indian
oceanic mantle via lower continental crust delamination
(Escrig
et al.,
2004; Hanan
et al.,
2004; Kamenetsky,
2001). This scenario is
only likely if the present southern Pacific is the
site of Rodinia fragmentation. It remains a tentative
model requiring further testing.
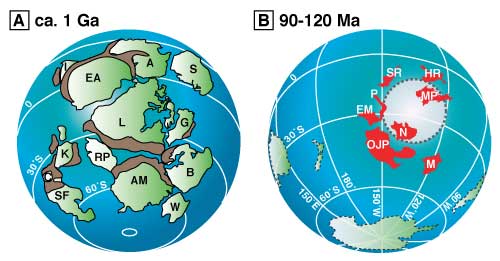
Figure 5: Paleogeographic reconstruction
models of Rodinia at 1010 Ma (A; Weil
et al., 1998)
and Cretaceous oceanic plateaus during the period
90-120 Ma (B; Lawver
et al., 2003). Present locations
of continents and Pacific superswell region are encompassed
by thin and thick dashed lines, respectively. A=Australia;
B=Baltica; C=Congo; EA=East Antarctica; G=Greenland;
I=India; K=Kalahari; L=Laurentia; RP=Rio de la Plata;
S=Siberia; SF=São Francisco; W=West Africa.
EM=East Mariana Basin; HR=Hess Rise; M=Manihiki Plateau;
MP=Mid-Pacific Mountains; N=Nauru Basin; OJP=Ontong
Java Plateau; P=Pigafetta Basin.
An alternative scenario is that the pyroxenites might
have been derived from oceanic crustal protoliths comprising
basaltic igneous crust and overlying sediment, because
such materials would be the volumetrically dominant
crustal input. A major drawback of this scenario is
that inferred granulite-grade differentiation is apparently
incompatible with the low geotherms typical of subduction
zones, where elemental fractionation via dehydration
can take place. The low-HFSE signature in the QGC may
preclude its derivation from a dehydrated slab because
it selectively retains HFSE due to their lower mobility
in slab-derived fluids and/or strong affinity for a
residual Ti-rich phase (e.g., Pearce & Peate,
1995). However, it has been suggested that the higher
geothermal gradients in the Precambrian were not conducive
to generating the stability field for blueschists even
in a subduction zone setting (Grambling,
1981; Maruyama & Liou,
1998). If the hypothesis of secular cooling is valid,
Proterozoic subducting slabs with high geothermal gradients
might have frequently undergone elemental redistribution
by partial anatexis.
Lower continental crust-like pyroxenite signatures
therefore do not allow us to rule out strictly their
derivation from ancient subducted oceanic crust. Nevertheless,
they suggest that long-term recycled eclogite/pyroxenite
heterogeneity was introduced in Pacific deep mantle
through Neoproterozoic amalgamation/breakup of the
Rodinia supercontinent, and that this heterogeneity
played a substantial role in the formation of the Ontong
Java Plateau as the surface expression of a large melting
anomaly in the Cretaceous Pacific mantle.
3.2 Implications for
Ontong Java Plateau magmatism
The origin of the Cretaceous oceanic plateaus (Figure
5B) that are widespread in the present-day western
Pacific, is the subject of a major debate in terms
of whether or not the melting was associated with high-temperature
mantle plumes. In this regard, two "endmember
scenarios" have been invoked to explain the generation
of the largest–the Ontong Java Plateau:
- High-temperature melting of a peridotite mantle
plume (>1500°C TP; Fitton & Godard,
2004), or;
- Entrainment of eclogite-bearing mantle by passive
upwelling (~1300°C TP; Korenaga,
2005)
The
presence of recycled eclogite/pyroxenite in the
magma source demonstrated here is compatible with
the second scenario. However, trace-element and
isotopic data support a composite source for the
magmas rather than a pure eclogite/pyroxenite source.
Given the voluminous emplacement of magma in off-axis
mature seafloor, it is likely that mantle hotter
than normal was implicated in entrainment of recycled
eclogite/pyroxenite. Thus, we envisage generation
of the Ontong Java Plateau as due to upwelling
of a composite mantle plume, although a quantitative
estimate of the TP is clearly required
before a decisive conclusion can be reached. For
this purpose, geochemical modeling should be revisited,
taking into account the contribution of recycled
materials combined with reasonable extents of heterogeneity.
Finally, it is interesting to consider
whether such a plume initiated from the core-mantle
boundary as theoretically predicted. Experimental investigations
suggest that sinking basaltic crust, except for the
coldest slabs, are likely to be gravitationally trapped
at the base of the mantle transition zone (e.g., Litasov
et al.,
2004). It therefore appears that the granulite-like
precursor of the QGC is incompatible with its storage
in the lower mantle. Moreover, the notion of a primitive
mantle-like source previously invoked to explain the
geochemical signatures of the Ontong Java Plateau lavas
(Fitton & Godard, 2004; Parkinson
et al., 2002; Tejada et al.,
2004)
must be revaluated because the contribution of recycled
eclogite/pyroxenite is critical for this argument.
Thus, our results lend no support to the hypothesis
of plume initiation at the core-mantle boundary. However,
the plume hypothesis may explain the temporal coincidence
of surges in the creation of oceanic plateaus with
the Cretaceous magnetic superchron (Larson & Olson,
1991). Furthermore, mounting evidence from seismic
methods clearly indicates the occurrence of a large-scale
low-velocity anomaly in the lower mantle under the
superswell region of the south-central Pacific (e.g.,
Hager
et al.,
1985; Romanowicz & Gung,
2002), which is spatially related to the birth
place of Cretaceous oceanic plateaus (Figure 5B; Courtillot
et al.,
2003; Larson,
1991). Thus, on-going
research for geochemical evidence of lower mantle and
core interaction in plume-related materials is critical
to obtaining a complete understanding of the origin
of the low-velocity anomaly and its role in present-day
hotspots and ancient oceanic plateaus.
Acknowledgments
We thank Gillian Foulger for her invitation
and help to present this webpage.
References
- Allègre,
C.J. and Turcotte, D.L., 1986. Implications of
a two-component marble-cake mantle. Nature, 323,
123-127.
- Blichert-Toft,
J., Frey, F.A. and Albarède, F., 1999.
Hf isotope evidence for pelagic sediments in
the source of Hawaiian basalts, Science, 285,
879-882.
- Courtillot,
V., Davaille, A., Besse, J. and Stock, J., 2003.
Three distinct types of hotspots in the Earth's
mantle, Earth Planet. Sci. Lett., 205,
295-308.
- Eisele,
J., Sharma, M., Galer, S.J.G., Blichert-Toft,
J., Devey, C.W. and Hofmann, A.W., 2002. The
role of sediment recycling in EM-1 inferred from
Os, Pb, Hf, Nd, Sr isotope and trace element
systematics of the Pitcairn hotspot, Earth
Planet. Sci. Lett., 196,
197-212.
- Escrig,
S., Capmas, F., Dupré, B. and Allègre,
C.J., 2004. Osmium isotopic constraints on the nature
of the DUPAL anomaly form Indian mid-ocean-ridge basalts, Nature, 431,
59-63.
- Fitton, J.G.
and Godard, M., 2004. Origin and evolution of magmas
on the Ontong Java Plateau. In: J.G. Fitton, J.J.
Mahoney, P.J. Wallace and A.D. Saunders (Eds.), Origin
and evolution of the Ontong Java Plateau, Geological
Society of London, London, pp. 151-178.
- Grambling,
J.A., 1981. Pressures and temperatures in Precambrian
metamorphic rocks, Earth Planet. Sci. Lett., 53,
63-68.
- Hager,
B., Clayton, R.W., Richards, M.A., Comer, R.P.
and Dziewonski, A.M., 1985. Lower mantle heterogeneity,
dynamic topography and the geoid. Nature, 313,
541-545.
- Hanan,
B.B., Blichert-Toft, J., Pyle, D.G. and Christie,
D.M., 2004. Contrasting origins of the upper
mantle revealed by hafnium and lead isotopes
from the Southeast Indian Ridge, Nature, 432,
91-94.
- Hauri,
E.H., 1996. Major-element variability in the
Hawaiian mantle plume, Nature, 382,
415-419.
- Hirschmann,
M. and Stolper, E.M., 1996. A possible role for
garnet pyroxenite in the origin of the "garnet
signature" in MORB, Contrib. Mineral.
Petrol., 124, 185-208.
- Hoffman,
P.F., 1991. Did the breakout of Laurentia turn
Gondwana inside out? Science, 252,
1409-1412.
- Hofmann,
A.W., 1997. Mantle geochemistry: the message
from oceanic volcanism, Nature, 385,
219-229.
- Ishikawa,
A., Maruyama, S. and Komiya, T., 2004. Layered
lithospheric mantle beneath the Ontong Java Plateau:
Implications from xenoliths in alnöite,
Malaita, Solomon Islands, J. Petrol., 45,
2011-2044.
- Ishikawa,
A., Nakamura, E. and Mahoney, J.J., 2005. Jurassic
oceanic lithosphere beneath the southern Ontong
Java Plateau: evidence from xenoliths in alnöite,
Malaita, Solomon Islands, Geology, 33,
393-396.
- Jacob,
D.E., Bizimis, M. and Salters, V.J.M., 2005.
Lu-Hf and geochemical systematics of recycled
ancient oceanic crust: evidence from Roberts
Victor eclogites, Contrib. Mineral. Petrol., 148,
707-720.
- Johnson,
K.T.M., 1998. Experimental determination of partition
coefficients for rare earth and high-field-strength
elements between clinopyroxene, garnet and basaltic
melt at high pressure, Contrib. Mineral.
Petrol., 133, 60-68.
- Jull,
M. and Kelemen, P.B., 2001. On the conditions
for lower crustal convective instability, J.
Geophys. Res., 106, 6423-6446.
- Kamenetsky,
V.S., 2001. Remnants of Gondwanan continental
lithosphere in oceanic upper mantle: evidence
from the South Atlantic Ridge, Geology,
29, 243-246.
- Korenaga,
J., 2005. Why did not the Ontong Java Plateau form
subaerially?, Earth Planet. Sci. Lett., 234,
385-399.
- Kroenke,
L.W., Wessel, P. and Sterling, A., 2004. Motion
of the Ontong Java Plateau in the hotspot frame
of reference: 122 Ma-present. In: J.G. Fitton,
J.J. Mahoney, P.J. Wallace and A.D. Saunders (Eds.), Origin
and evolution of the Ontong Java Plateau, Geological
Society of London, London, pp. 9-20.
- Larson,
R.L., 1991. Latest pulse of Earth: evidence for
a mid-Cretaceous superplume, Geology, 19,
547-550.
- Larson,
R.L. and Olson, P., 1991. Mantle plumes control
magnetic reversal frequency, Earth Planet.
Sci. Lett., 107, 437-447.
- Lawver,
L.A., Dalziel, I.W.D., Gahagan, L.M., Martin,
K.M. and Campbell, D.A., 2003. The PLATES 2003
Atlas of Plate Reconstructions (750 Ma to Present
Day). PLATES Progress Report No. 280-0703 (UTIG
Technical Report No. 190), 97 pp.
- Litasov,
K., Ohtani, E., Suzuki, A., Kawazoe, T. and Funakoshi,
K., 2004. Absence of density crossover between
basalt and peridotite in the cold slabs passing
through 660 km discontinuity, Geophys. Res.
Lett., 31: doi:10.1029/2004GL021306.
- Maruyama,
S. and Liou, J.G., 1998. Initiation of ultrahigh-pressure
metamorphism and its significance on the Proterozoic-Phanerozoic
boundary, Island Arc, 7,
6-35.
- McDonough,
W.F. and Sun, S.-S., 1995. The composition of
the Earth, Chem. Geol., 120,
223-253.
- Meert,
J.G. and Torsvik, T.H., 2003. The making and
unmaking of a supercontinent: Rodinia revisited, Tectonophysics, 375,
261-288.
- Parkinson,
I.J., Schaefer, B.F. and Arculus, R.J., 2002.
A lower mantle origin for the world's biggest
LIP? A high precision Os isotope isochron from
Ontong Java Plateau basalts drilled on ODP Leg
192, Geochim. Cosmochim. Acta, 66, Supplement,
A580.
- Pearce,
J.A. and Peate, D.W., 1995. Tectonic implications
of the composition of volcanic arc magmas, Annu.
Rev. Earth Planet. Sci., 23,
251-285.
- Pearson,
D.G. and Nowell, G.M., 2004. Re-Os and Lu-Hf
isotope constraints on the origin and age of
pyroxenites from the Beni Bousera peridotite
massif: implications for mixed peridotite-pyroxenite
mantle sources, J. Petrol., 45,
439-455.
- Pertermann,
M. and Hirschmann, M., 2002. Trace-element partitioning
between vacancy-rich eclogitic clinopyroxene
and silicate melt, Am. Mineral., 87,
1365-1376.
- Romanowicz,
B. and Gung, Y., 2002. Superplumes from the core-mantle
boundary to the lithosphere: implications for
heat flux, Science, 296,
513-516.
- Rudnick,
R.L. and Goldstein, S.L., 1990. The Pb isotopic compositions
of lower crustal xenoliths and the evolution of lower
crustal Pb, Earth Planet. Sci. Lett., 98,
192-207.
- Rudnick,
R.L. and Taylor, S.R., 1987. The composition and
petrogenesis of the lower crust: a xenolith study, J.
Geophys. Res., 92, 13981-14005.
- Schmitz,
M.D., Vervoort, J.D., Bowring, S.A. and Patchett,
P.J., 2004. Decoupling of the Lu-Hf and Sm-Nd
isotope systems during the evolution of granulitic
lower crust beneath southern Africa, Geology, 405,
405-408.
- Stacey,
J.S. and Kramers, J.D., 1975. Approximation of
terrestrial lead isotope evolution by a two-stage
model, Earth Planet. Sci. Lett., 26,
207-221.
- Stracke,
A., Bizimis, M. and Salters, V.J.M., 2003. Recycling
oceanic crust: quantitative constraints, Geochem.
Geophys. Geosys., 4, doi:10.1029/2001GC000223.
- Tejada, M.L.G., Mahoney, J.J., Castillo, P.R.,
Ingle, S., Sheth, H.C. and Weis, D., 2004. Pin-Pricking
the Elephant: evidence on the origin of the Ontong
Java Plateau from Pb-Sr-Hf-Nd isotopic characteristics
of ODP Leg 192 basalts. In: J.G. Fitton, J.J. Mahoney,
P.J. Wallace and A.D. Saunders (Eds.), Origin
and evolution of the Ontong Java Plateau, Geological
Society of London, London, pp. 133-150.
- Tejada,
M.L.G., Mahoney, J.J., Neal, C.R., Duncan, R.A.
and Petterson, M.G., 2002. Basement geochemistry
and geochronology of central Malaita, Solomon
Islands, with implications for the origin and
evolution of the Ontong Java Plateau, J.
Petrol., 43, 449-484.
- Vervoort,
J.D., Patchett, P.J., Albarède, F., Blichert-Toft,
J., Rudnick, R.L. and Downes, H., 2000. Hf-Nd
isotopic evolution of the lower crust, Earth
Planet. Sci. Lett., 181,
115-129.
- Vervoort,
J.D., Patchett, P.J., Blichert-Toft, J. and Albarède,
F., 1999. Relationships between Lu-Hf and Sm-Nd
isotopic systems in the global sedimentary system, Earth
Planet. Sci. Lett., 168,
79-99.
- Watson,
E.B., Ben Othman, D., Luck, J.-M. and Hofmann,
A.W., 1987. Partitioning of U, Pb, Cs, Yb, Hf,
Re and Os between chromian diopsidic pyroxene
and haplobasaltic liquid, Chem. Geol., 62,
191-208.
- Weil,
A.B., Van der Voo, R., Niocaill, C.M. and Meert, J.G.,
1998. The Proterozoic supercontinent Rodinia: paleomagnetically
derived reconstructions for 1100 to 800 Ma, Earth
Planet. Sci. Lett., 154, 13-24.
last updated 3rd
September, 2007 |