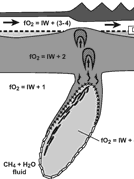 |
The Genesis of Basaltic Magmas, the Petrology of the Upper Mantle, and the Nature of the Lithosphere/Asthenosphere Boundary |
David H. Green
Earth Sciences, School of Physical Sciences, University of Tasmania, Hobart, Tasmania, 7005, Australia, david.h.green@utas.edu.au
Full publication list, David H. Green
This web page summarizes two review papers arising from the presentation of the International Mineralogical Association (IMA) Medal and lecture at the European Mineralogical Societies Conference in Frankfurt, Germany in 2012 and the presentation of the Shensu Sun memorial lecture at the annual Chinese Conference on Geochemistry and Geodynamics held at Lanzhou University in 2012, and also given at other Chinese universities. The expansion from lectures to publication resulted in two papers with some overlap and re-use of illustrations:
- the IMA Medal lecture emphasised the ‘forward’ approach to magma genesis, i.e. subsolidus phase relations and melting of peridotite. This lecture gave rise to the paper: Green, D.H. (2015) Experimental petrology of peridotites, including effects of water and carbon on melting in the Earth’s upper mantle. Physics and Chemistry of Minerals. 42, 95-102. DOI 10.1007/s00269-014-0729-2
- the Shensu Sun Memorial paper emphasised the ‘inverse’ approach, i.e. the high pressure liquidus phases of mantle-derived magmas, their liquidus temperatures and solution of volatiles, particularly water and carbonate. This Memorial paper gave rise to the publication: Green, D.H. & Falloon, T.J. (2015) Mantle-derived magmas: intraplate, hot-spots and mid-ocean ridges. Science Bulletin 60 (22), 1873–1900. www.scibull.com DOI 10.1007/s11434-015-0920-y
A common theme throughout these experimental works is the parallel study of the petrology and geochemistry (major, minor and trace elements) of natural Alpine-type ophiolites, eclogites, mantle xenoliths and mantle-derived peridotite (i.e. with high pressure,mineral parageneses) and of natural primary magmas (i.e. segregated from residual peridotite) and derivative magmas (i.e., with composition variably modified by crystallization processes). The material was largely drawn from high-pressure experimental investigations carried out at the University of Tasmania and the Australian National University from 1962 to 2012 by the author, research fellows, Ph.D. students and visitors, with excellent technical and professional support.
Experimental studies established phase relations, including melts and fluids, constraining upper mantle petrogenesis and geodynamics. Of particular importance is the role of small water and carbon content in controlling the solidus and stabilizing pargasitic amphibole in fertile lherzolite to depths of 90-100 km. The phase relations of lherzolite, including melting behaviour, provide explanations for the lithosphere and asthenosphere. They also provide explanations for distinctive magmatism at passive plate margins (MORB), intraplate settings (OIB and rifts), and above subduction zones. In the context of plate tectonics, petrology constrains models of temperature, rheology, buoyancy, electrical conductivity and seismic properties.
For over 50 years, the use of high-pressure piston/cylinder apparati, combined with an increasing diversity of microbeam analytical techniques, has enabled the study of mantle peridotite compositions and magmas derived by upper mantle melting. The experimental studies have been guided by the petrology and geochemistry of peridotites from diverse settings and by the remarkable range of mantle-derived magma types. The inferred composition of the upper mantle, based on ‘fertile’ or least refractory compositions among natural high pressure peridotites is lherzolite with 3-4 wt% of Al2O3 and CaO, and 0.2-0.4 wt% Na2O. From studies of both magmas and peridotites, a further characterisation of lherzolites is as ‘fertile’ (MORB-source), ‘enriched’ (OIB-source) or ‘depleted’ (in High Field Strength Elements (HFSE), Light Rare Earth Elements (LREE) and Large Ion Lithophile Elements (LILE)). On this basis, model mantle compositions:
- Fertile: MPY (MOR Pyrolite) and HZ (Model MORB-source mantle of Hart & Zindler, 1986),
- Enriched: HPY (Hawaiian pyrolite), and
- Depleted : Tinaquillo lherzolite
have been studied experimentally at high pressure (0.2 to 6 GPa) and high temperature to determine their mineralogy, solidus temperatures and melt + residue compositions. Initial studies were under dry conditions, then with varying H2O contents. The exploration of the role of carbon in the upper mantle necessitated the control of oxidation state as carbon occurs as CO2, (CO3)-2, C or CH4.
Experiments defined the solidus and melting behaviour of lherzolites (HPY particularly) with carbonate (CO3)=, or CO2, (with and without H2O); with graphite+(H2O-rich fluid); and with graphite+[(CH4-H2O) fluid]. A simple system analogue (Nepheline+Forsterite+Quartz) was studied to clarify the effects of oxidation state, water and carbon on the temperature of the solidus and on the composition of melts lying on the Ol+Opx cotectic.
Experimental studies established a major role for pargasite as a hydrous mineral stable to the solidus of fertile lherzolites at < 3 GPa resulting in an explanation for the lithosphere/asthenosphere boundary and for distinctive intraplate and MOR basalts. However the recognition that nominally anhydrous minerals (NAMs – olivine, orthopyroxene, clinopyroxene and garnet) may contain significant water contents at high pressure led to alternative models in which, for MORB-source mantle particularly, water is contained in NAMs and pargasite stability does not play a significant role. In contrast to the distinctive P-T shape of the pargasite-lherzolite dehydration solidus, in this alternative model, water is treated as a dilute solid solution in NAMs and the modelled lherzolite solidus parallels the dry solidus with positive dT/dP and liquidus depression dependent on partition between NAMs and melt.
To resolve this debate, experiments with variable, controlled water contents in fertile lherzolite sandwiched between olivine layers allowed direct determination of water content in olivine, pargasite stability, presence of partial melt or hydrous fluid, and of mineral compositions – all in the same experiment. The use of FTIR spectroscopy to monitor water content of the monomineralic layers has shown that, at ~1,000°C, fertile lherzolite (MORB-source upper mantle) can store ~200 ppm H2O in defect sites in nominally anhydrous minerals (olivine, pyroxenes, garnet and spinel). A water content of > 100 ppm approx. at P < 3 GPa, stabilizes amphibole (pargasite) to the lherzolite solidus. However, at P > 3 GPa, water in excess of ~200 ppm appears as an aqueous vapour phase and this depresses the temperature of the upper mantle solidus. Provided the uppermost mantle (lithosphere) has 100 ppm < H2O < 4,000 ppm, the mantle solidus has a distinctive P-T shape. The temperature of the vapour-undersaturated or dehydration solidus is approximately constant at 1,100°C at pressures from ~0.5 to ~3 GPa and then decreases sharply to ~1,010°C at > 3 GPa. The intersection between an oceanic intraplate geotherm and the vapour-undersaturated solidus (hydrous and carbonate-bearing silicate melt) of lherzolite at ~3 GPa produces a strong rheological effect and marks the lithosphere/asthenosphere boundary. The presence of incipient melting sharply reduces viscosity at ~90 km depth and gives rise to the asthenosphere between ~90 km and ~250 km depth. The base of the asthenosphere near 250 km may be the intersection of the geotherm with the solidus of lherzolite + (H2O + CH4), i.e. approaching the dry solidus.
The preceding discussion and Green (2015) largely focus on ‘forward experiments’ i.e. on lherzolite melting. The complementary approach is commonly referred to as the ‘inverse’ approach and is emphasized in Green & Falloon (2015). In this approach, potential primary, mantle-derived magmas are selected using the most magnesian glass and olivine phenocryst compositions in basaltic magmas and also the suite of intraplate magmas which contain spinel or garnet peridotite xenoliths i.e. of high-pressure origin. The liquidus phases of the potential primary magma have been determined as functions of pressure and temperature, seeking conditions where olivine + orthopyroxene ± clinopyroxene ± garnet ± spinel are liquidus phases. Under C- and H-absent conditions, only olivine tholeiites to alkali olivine basalts have Ol + Opx ± Cpx as high-pressure liquidus phases. Addition of H2O accessed olivine basanites at 2.5–3 GPa, ~1200°C, but both CO2 and H2O were necessary to obtain saturation with Ol, Opx, Cpx and Ga at 2.5–3.5 GPa for olivine nephelinite and olivine melilitite.
The forward and inverse experimental studies are combined to formulate a petrogenetic grid for intraplate, ‘hot-spot’ and MOR magmatism within the plate tectonics paradigm. The asthenosphere is geochemically zoned by slow upward migration of incipient melt. The solidus and phase stabilities of lherzolite with low water contents (< 4,000 ppm) create the rheological properties enabling the thin plate behaviour of the oceanic lithosphere and thus the plate tectonics expression of Earth’s convection. Some authors infer a second type of convection by deep mantle thermal plumes from the core-mantle boundary (‘hot-spot’ volcanism), expressed as linear volcanic chains with sources ‘fixed’ relative to plate movement, high melt production and compositional heterogeneity. A cornerstone of this hypothesis is the high temperature required of deep thermal thermal plumes, with potential temperature (Tp) differing by ~200°C above normal MORB-source mantle. However, the temperatures and pressures required for derivation of the parental magmas at mid-ocean ridges and ‘hot-spots’ respectively are the same within error (Tp ~1430°C and 1.5 – 2 GPa). It is argued that there is no evidence from the study of parental magmas to support the ‘deep mantle thermal plume’ hypothesis. The preferred alternative is the presence of old subducted slabs, relatively buoyant and oxidised and relatively C-rich with respect to MORB source mantle. These composional heterogeneities are suspended in or below the lower asthenosphere and thus decoupled from overlying plate movement (Figure 3).
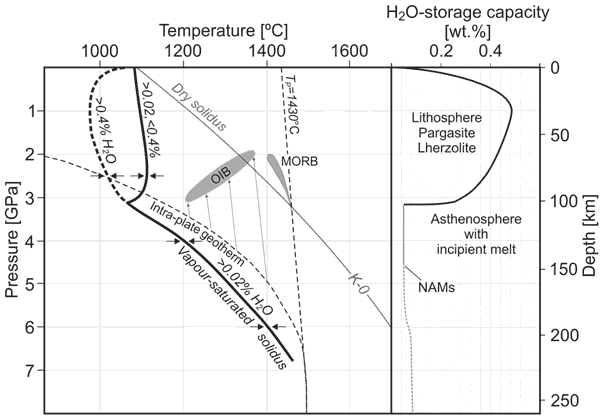
Figure 1: Summary of the experimental determination of solidi for the model mantle composition (HZ) and of the stability of pargasite, using varying water contents and simultaneously determining the water contained in olivine, orthopyroxene and clinopyroxene.
Right panel plots the water storage capacity of fertile, MORB-source mantle (i.e. MPY or HZ Lherzolite) as a function of depth, along the vapour saturated solidus. Shallower than ~100 km depth, pargasite is stable to the solidus but the modal abundance of pargasite reaches a maximum at 1-1.5 GPa and decreases towards higher pressure. Thus the water storage capacity decreases with increasing pressure, controlled by modal pargasite. Pargasite becomes unstable at > 3 GPa, and water storage capacity drops to that which can be retained in NAMs in fertile lherzolite (~200 ppm).
Left panel shows experimentally determined solidi (‘Dry’ or C and H absent; small C and H contents), together with a model intraplate geotherm (short-dashed line) and mantle adiabat (Tp = 1430°C). The intraplate geotherm intersects the mantle dehydration solidus (pargasite-bearing lherzolite) at ~90 km and at deeper levels a small melt fraction is present if water contents are >200 ppm (a region of ‘incipient melting’). This provides an explanation for the asthenosphere and the lithosphere/asthenosphere boundary. The geotherm is shown joining the mantle adiabat at 200-250 km. The depth interval from 90 to 250 km is one in which a very small near-solidus melt may migrate along the geotherm, having little effect on the geotherm.but a large effect on trace element contents. This carbonated hydrous silicate melt is equilibrated with garnet lherzolite and highly enriched in LILE and LREE. Over time they enrich the upper asthenosphere and deplete the lower asthenosphere in incompatible elements. Near-adiabatic upwelling (diapirs or thermal instabilities) from within the low viscosity asthenosphere result in locally perturbed geotherms and increasing melt fraction within the upwelling mantle. MORB are shown as sourced from upwelling lower asthenosphere i.e. source lherzolite with ~200 ppm H2O, depleted LREE and LILE element patterns.
Intraplate basalts, including OIB, are shown as formed by upwelling of enriched lherzolite from the middle and upper asthenosphere as a consequence of lithospheric stretching and thinning, the super-adiabatic gradient in the lithosphere and asthenosphere and the increasing temperature, buoyancy and rheology differences between diapir and lithosphere. Near-adiabatic temperatures within the diapir (fine dotted lines) and magma extraction aided by degassing C-rich fluid at 1.3 to 2 GPa,add complexity to intraplate and ‘hot-spot basalts. Intraplate magmas range from olivine melilitites and nephelinites at deeper levels and smaller melt fraction, to olivine tholeiites with higher melt fraction and higher temperatures. Small carbon contents, dissolved as (CO3)= in the near-solidus melts, are necessary for generating mantle-derived oivine melilitites and olivine nephelinites.
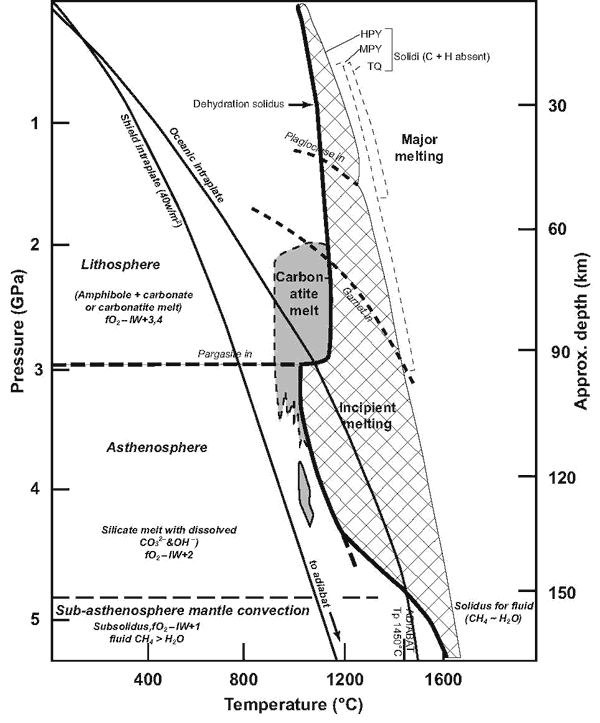
Figure 2: A model for the Earth’s uppermost mantle in intraplate locations emphasizing young continental or oceanic crust and lithosphere.
The model is based on experimental determination of phase assemblages, solidus and melt compositions of lherzolite and assumes minor carbon and hydrogen (water) in the upper mantle. The model also suggests decreasing oxygen fugacity with increasing depth. Pargasite-bearing spinel lherzolite overlying pargasite-bearing garnet lherzolite (with CO2-rich fluid inclusions) characterize the uppermost mantle above the decarbonation boundary (Ol+Cpx+CO2<–>Opx+Dol) at ~ 2 GPa. A field for carbonatite melt is shown, particularly for perturbed geotherms, but it is suggested that this melt is very mobile with low density and viscosity, even at very small melt fraction. Carbonatite melt may migrate through the asthenosphere along the geotherm to the dolomite-bearing lherzolite solidus. Alternatively, migration along a perturbed geotherm intersects the decarbonation solidus (P~2 GPa, 950°C < T < 1100°C) where olivine and clinopyroxene are precipitated and CO2-rich vapour released. If this is so, then a sodic, dolomitic carbonatite melt in pargasite lherzolite may be a transient feature at ~60–90 km beneath young (?< 1 Ma) intraplate volcanic provinces, i.e. along a currently perturbed geotherm.
Conditions along an unperturbed intraplate geotherm are subsolidus (with respect to hydrous silicate melt) to ~90 km defining the lithosphere but at this depth the geotherm enters a P-T field for incipient melting (the asthenosphere), the melt being a carbonate-bearing olivine melilite nephelinite to olivine melilite. The depth of the lithosphere/asthenosphere boundary is stable against T variation of ~100°C due to the strong negative dT/dP of the pargasite breakdown (dehydration solidus). The lower boundary of the asthenosphere is assumed to be the second intersection of the geotherm and lherzolite solidus which will be dependent on the CH4/H2O of the C-H-O fluid.
Slow upward migration of the near-solidus hydrous silicate melt within the asthenosphere produces a depth interval of enrichment (upper) and depletion (lower) in incompatible elements, particularly H, C, P, K, LILE and LREE. Consequently there is a decoupling of major elements and incompatible trace elements.
The figure illustrates the argument that the phase relationships and melting behaviour of fertile or enriched lherzolite are a necessary and sufficient condition for the lithosphere/asthenosphere boundary and enable the plate tectonic behaviour of the Earth.
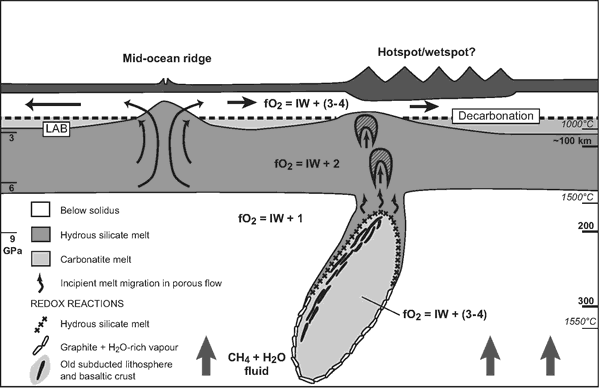
Figure 3: Diagram suggesting a model for intraplate ‘hot-spot’ or island chain volcanism, in which the cause of the volcanism is ‘fixed’ relative to plate movement.
The model attributes the cause of the long-lived melting anomaly producing island chains or ‘hot-spot tracks' to compositional heterogeneity within the mantle beneath or within the lowermost part of the asthenosphere. Specifically, the compositional heterogeneity is suggested to be old subducted slab(s), mainly of refractory harzburgite and metamorphosed oceanic crust. The interface between such slabs (fO2 ~ IW+3-4 log units, high Fe+++/Fe++ and carbonate-bearing) and ambient mantle (fO2 ~ IW+1-2 log units, graphite- or diamond-bearing, sulphide-bearing and low Fe+++/Fe++) is a redox contrast at which fH2O is a maximum in C,H,O fluid, triggering melting at the water-saturated solidus or (at deeper levels) graphite/diamond and water-rich fluid. The near-solidus melts are depicted as lowering viscosity to permit diapirism in which mixing and reaction (including redox melting) occurs between asthenospheric mantle and old subducted crust + lithosphere. Melt segregation within upwelling mantle occurs at shallower depths and higher melt fraction. Although the model suggests a temperature anomaly (‘plume’ or diapir) at shallow depths in lithosphere and asthenosphere, there is no deep-seated thermal plume (ΔTp ~200°C) and the melting anomaly is effectively due to compositional rather than temperature heterogeneity in the mantle. Magmas are enriched relative to MORB in (C, H, O, P, K and incompatible trace element) contents. Isotopic and chemical heterogeneity reflects the old subducted slab/ambient mantle interface.
References
last updated 1st September, 2016 |