 |
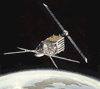
ERS-1
|
Sea-floor
spreading and deformation processes in the
South Atlantic Ocean:
Are hot spots needed?
|
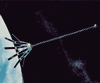
GEOSAT |
|
|
1.
Introduction
The publication of the SEASAT satellite-derived
gravity map of the world’s oceans in the mid
1980s, with resolution of ~ 60 km and greater, by
Haxby revealed for the first time the global fabric
of plate tectonics, and resulted in a major advance
in our understanding of Earth processes. In the mid
1990s Sandwell & Smith (1997) further
improved the resolution of the satellite-derived gravity
field using the combined geodetic mission data of
GEOSAT and ERS-1 giving a resolution 30-40 km and
greater. The enhanced detail of plate tectonic processes
provided by these new data did not, however, result
in such a dramatic advance as would have been expected.
Hot spots and mantle plumes were by then firmly established
concepts in explaining the observed linear chains
of volcanic islands and in providing a super-deep
mantle frame of reference (the hotspot reference frame)
that was independent of plate motions at the surface.
Accumulating scientific evidence
over half a decade and recent GPS-derived absolute
plate motions suggest that both the mantle plume frame
of reference and the age dependence along volcanic
lineaments are not as strong as once believed. This
article investigates the complex tectono-magmatic
processes involved in the opening of the Central,
Equatorial and South Atlantic Ocean and asks the simple
question of whether there is an alternative tectonic
model to explain the phenomena seen in the satellite
gravity field. The case is made that hot spots or
mantle plumes are not necessarily required to explain
the volcanic lineaments and that most, if not all,
features result from deformational processes during
the evolution of the plates. Such a model has a range
of implications which, hopefully, can be tested to
further refine the model.
This study uses throughout the satellite
data of Sandwell & Smith, which have
resolution of 30-40 km and greater (Sandwell,
2002). To evaluate and develop the proposed model
the resolution of the satellite gravity needs to be
increased to about 10 km. This satellite resolution
has been achieved using GEOSAT and ERS-1 geodetic
mission data by innovative research and development
funded under a ROPA award to the University of Leeds
(1996-1998; to Prof. Derek Fairhead) and more recently,
via Leeds University spin-off company GETECH
of which Professor Fairhead is the CEO, by conducting
two oil industry consortium studies to prove the technology
and to implement it to map the world’s continental
margins (Maus et al., 1998; Fairhead
et al., 2001a,b; Green & Fairhead,
1998). The need for such resolution is illustrated
in this study and will significantly improve our understanding
of oceanic crust-forming processes, particularly the
role of magmatism and intra-plate deformation,
|
2. The
South Atlantic Ocean
Our current knowledge, based on existing
research, indicates that slow-spreading mid-ocean
ridges such as the mid-Atlantic ridge are
strongly segmented along their axes by transform
faults (Figures 1 & 2).
|
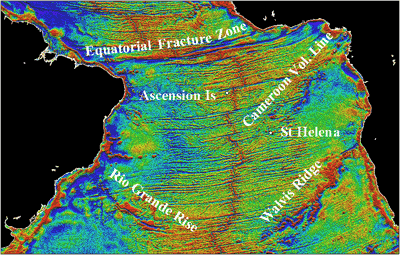
Figure 1: The satellite free air gravity
of the Central, Equatorial and South Atlantic
Oceans. Click on image for a larger version.
|
In the South
Atlantic these transform faults are typically
spaced some 50-100 km apart, reflect relative
plate motion directions of the newly formed
crust and occur at offsets of the normal faulted
median rift valley that marks the axis of the
ridge. The sites of these active transforms
are regions of decreased magma generation, resulting
in the transform zone being starved of volcanism,
which is expressed as a deep trough in the oceanic
crust. |
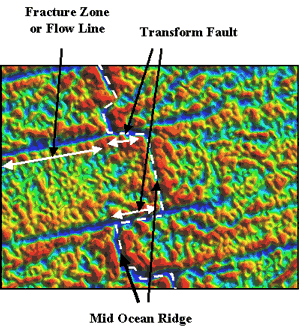
Figure 2: Free air gravity
Image of a small part of the mid South Atlantic
mid-ocean ridge. |
At greater distance from
the ridge crest, transform motion ceases. This
change occurs at the adjacent ridge offset;
between the ridge offsets the transform separates
different plates, while beyond the ridge offsets
the transform separates older (or younger) crust
belonging to the same plate. Beyond this transition
the transform fault is referred to as a fracture
zone or flow line. The starved nature of the
flow line and the differing age of the oceanic
crust across it generates a distinct bathymetric
and gravity feature (Figure 2) that can be traced
for large distances away from the ridge axis
and preserves evidence of former plate tectonic
processes and movement vectors. |
|
The hotspot concept for the evolution of the South
Atlantic has generally been explained by active hotspot/mantle
plumes being located along or close to the ridge axis,
generating trails of volcanic islands and seamounts
as the plate migrates over the hotspot. These hotspot
trails strike at oblique angles to the flowlines suggesting
deeper processes are in operation than those that
formed the flowlines. These hotspots are traditionally
linked to upwelling convective instabilities or “mantle
plumes”, originating from thermal boundary layers
at the base of the upper mantle (the 670 km discontinuity)
or even the core-mantle boundary at 2,900 km depth.
Assuming the hotspot reference frame is valid, the
seamount trails would then provide an important record
of past motion of the African plate.
|
Within the South Atlantic there are a number of distinct
volcanic lineaments (Figure 1) in the form of aseismic
ridges (e.g. Rio Grande Rise, Walvis Ridge) and chains
of seamounts and oceanic islands (e.g. St. Helena
Seamounts and the Cameroon Volcanic Line). These volcanic
lineaments have been previously explained by the upwelling
of deep mantle plumes since the onset of continental
break-up in the Early Cretaceous – the St. Helena
Seamount chain and the Walvis Ridge linked to the
St. Helena and Tristan mantle plumes respectively
(Wilson, 1992). The simplicity of the mantle
plume and hotspot trails concept tends to raise more
questions than it answers.
-
When St. Helena should be the
youngest volcanic centre, why should there be so
much Tertiary-Present volcanic activity occurring
within the Cameroon Volcanic Line (Figure 1) where
it straddles the African continental margin?
-
The Walvis Ridge extends from
the continental margin of Africa to the active volcanic
islands of Tristan da Cunha and Gough. The SW end
of the hotspot trail consists of a ~ 400 km wide
region of scattered seamounts, small ridges and
islands, similar in morphology to that of the St.
Helena Chain. Why does the conjugate Rio Grande
Rise on the South American plate, exhibits a total
different morphology?
-
Why does the Cameroon Volcanic
Line not have a conjugate volcanic lineament on
the South American plate?
-
Why should the South Atlantic
plumes track the mid-oceanic ridge when the hotspot
reference frame is independent of plate tectonics?
If we can establish the processes
which form these trails of South Atlantic volcanism,
then we can gain important insights into the role of
mantle plumes or other mechanisms in continental break-up
and the subsequent evolution of the ocean basins. |
3.
The Central and Equatorial Atlantic
The Central Atlantic is located to
the north of the Equatorial fracture zones that dominate
the bathymetric morphology and satellite gravity field
between NE Brazil and the Gulf of Guinea (Figure 1).
The Central Atlantic Ocean opened in the early Mesozoic
separating Europe/Africa from North America (Wilson,
1997), whereas the South Atlantic Ocean opened later
in the early Cretaceous and propagated northwards. The
joining of these two independent spreading centres in
the early-mid Cretaceous resulted in a major shear zone
developing between West Africa and the northern margin
of Brazil. Since the opening of these two spreading
systems was different, differential motion was taken
up as deformation predominantly in Africa and the Caribbean
and least in South America. The change in flow line
geometry north and south of the Equatorial fracture
zones is shown in Figures 3, 4 and 5.
Figure 3 shows clearly defined flow
lines with isochron ages for the Central Atlantic. Here
the flow lines exhibit clear changes in direction indicating
that the relative movement of the plates has changed
due to plate interactions elsewhere on the globe e.g.
India colliding with Asia and Africa colliding with
Europe, resulting in the need for global adjustments
in plate motions. Figure 4 shows the same isochrons
for the northernmost part of the south Atlantic. When
the flow lines and isochrons of Figures 3 and 4 are
displayed together in Figure 5 there is immediate recognition
that the flow lines are responding differently e.g.
the curvature of the flow lines (marked as ‘+’
and ‘–’) is opposite to the north
and south of the Equatorial fracture zone. This implies
there are significant differences in relative plate
motions to the north and south of the Equatorial fracture
zone and this has resulted in deformation propagating
into the Caribbean and Africa. Motion between Africa
and Eurasia is shown in a different way in Figure 6
by tracking the motion of Africa with respect to Eurasia.
The motion has been such that the tracks increase in
length from northwest to east Africa. The amplification
of the plate motion shows clear changes in plate motion
at about 84, 65 and 37 Ma. Arrows indicate these changes
for the movement of the Indian plate.
|
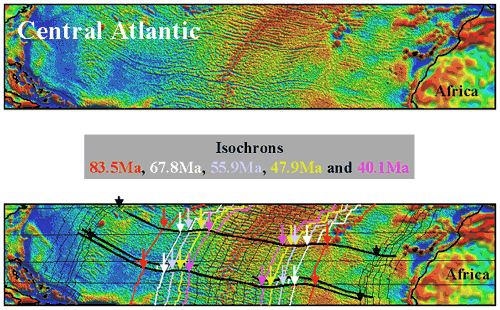
Figure 3: Free air gravity anomaly
of the central Atlantic with flow lines and isochrons
superimposed on the lower diagram. |
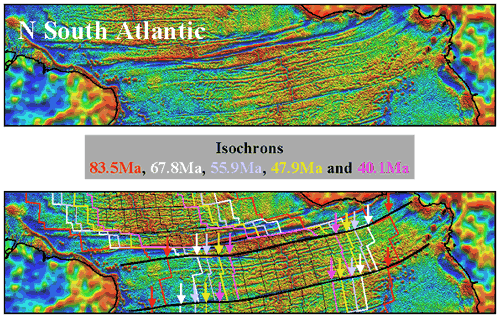
Figure 4: Free air gravity anomaly
of the northern South Atlantic with flow lines and
isochrons superimposed on the lower diagram.
|
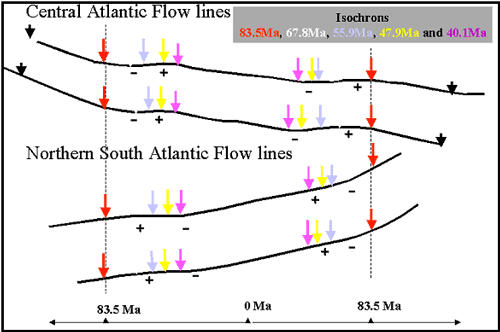
Figure 5: Comparison of the flow lines shown
in Figures 3 & 4 (normalised to
isochron 83.5 Ma) showing the distinct changes in
relative plate motion highlighted
by the ‘+’ and ‘–’ curvature
signs.
|
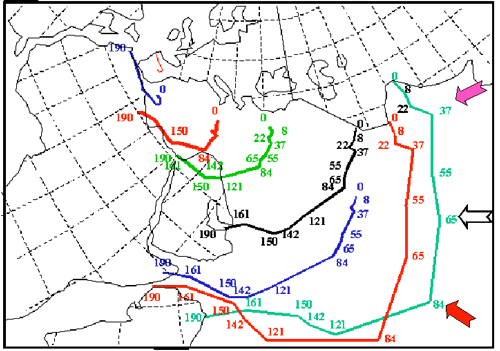
Figure 6: Relative plate motion between Africa
and Eurasia and between India and Eurasia.
|
In Africa, this differential movement resulted in
the development of major passive extensional structures
(basin formation) and shear deformation (Figure 7).
The stratigraphy of these internal African basins
is a more sensitive indicator of stress changes. Detailed
geological studies within the southern Chad basins
reveals the poly-phase tectonic development of these
basins, all affected in different ways, due to basin
orientation, to changes in plate stress.
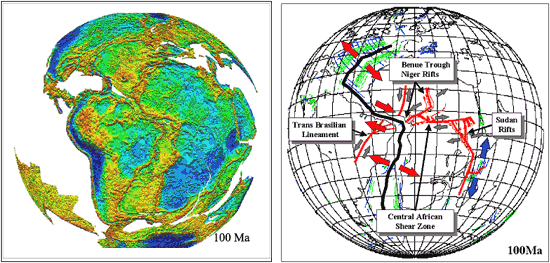
Figure 7: Plate reconstruction
at 100 Ma using gravity data onshore and offshore
and the associated deformation models for Africa and
South America. Click on image to enlarge.
|
The principal stratigraphic
events marked by arrows in Figure 8 indicate those events
seen in the fabric of the ocean crust. Clearly the basin
stratigraphy is more sensitive to smaller scale tectonic/stress
change events than the ocean crust. Major inequalities
in plate motion are expressed within the complex fabric
of the oceanic crust. If the definition of the fabric
was better resolved by having higher-resolution satellite
gravity data then there is a real possibility that more
of the complexity could be unravelled in terms of evolving
plate motions and deformation that should link temporally
and spatially with tectonics and deformation events
within the rift basins of Africa as recorded in the
stratigraphy and deformation of the sedimentary basins
(Fairhead & Green, 1989; Fairhead &
Binks, 1991: Fairhead & Guiraud, 2002).
The stratigraphy of the basins clearly indicates the
events we should be looking for in the ocean basins.
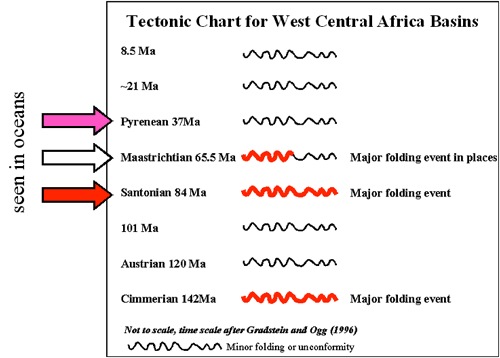
Figure 8: Generalised tectonic Chart
for West Central Africa based on extensive geological
and seismic interpretation. The arrows show the tectonic
events seen in the fabric of the ocean crust. |
4.
Hypotheses that need testing
We have demonstrated above that changes
in relative plate motion have resulted in stress changes
in Africa that have been responsible for the development
of a major rift basin system cutting Africa from Nigeria
via Lake Chad to Algeria and from Cameroon through southern
Chad, northern Central African Republic into Sudan and
Kenya (Figure 7). New data for global absolute plate
motions based on plate tectonic (NUVEL 1A), GPS and
astronomical studies (ITRF97) (Figure 9) indicate that
the African plate is presently moving to the NE whereas
the South American plate, east of the Andes, is moving
to the NW. These directions of motion are consistent
within both oceanic and continental domains. For example,
within the South Atlantic Ocean the oceanic island of
Ascension (Figure 1) lies just to the west of the mid-Atlantic
ridge and moves along the same vector as South America,
whereas the oceanic island of Gough (goug in Figure
9) lies to the east of the ridge axis and moves with
the African plate.
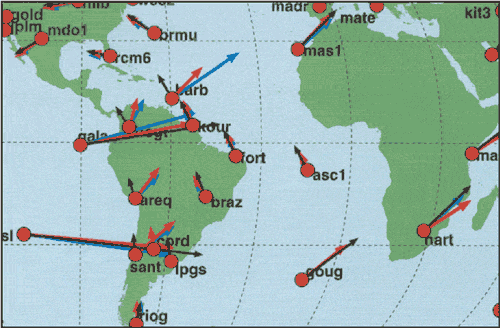
Figure 9: Absolute plate motions
based on GPS data (red arrows) from GFZ Potsdam, Germany
for the period 1993-2000 compared to directions of older
estimates of motion predicted by the NUVEL 1A model
(black arrows) and astronomic (blue arrows). |
If a vector
diagram of the African and South American absolute
motions is constructed (Figure 10) then the
relative motion between Africa and South America
is essentially E-W and this is what the transforms
and flow lines reflect. Slight changes in flow
line direction indicate changes in the relative
motion of the plates brought about by the consequence
of plate interactions/collisions elsewhere e.g.
Africa colliding with Europe and India colliding
with Asia. |
The South Atlantic ridge
axis will have an absolute motion equivalent
to the mean between the African and South American
vector directions. This dictates that the ridge
axis is moving close to due north. Thus magmatic
processes that are independent of, and deeper
than, the flow line geometry will be influenced
by absolute plate motions rather than relative
motions. Combining these phenomena, if data
resolution permits, will provide vector controls
on the absolute and relative plate motions at
any given time in the past. This has not been
previously exploited in unravelling past relative
and absolute plate motions. |
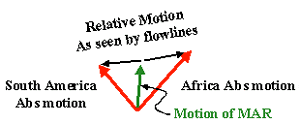
Figure 10: Vector diagram showing relations
between absolute plate motions, relative motions
and motion of the mid-ocean ridge (MAR) If
we know the relative motions and the motion
of the MAR then absolute motions can be deduced.
|
|
The absolute plate
motion results indicated in Figure 9 are based on the
simple assumption that there is no net-rotation of the
lithosphere (i.e. using a reference frame that yields
zero for the integral of v x r over
the Earth’s surface, where v is the plate
velocity at position r). The GFZ-Potsdam GPS
data (red arrows) are considered to be the most reliable.
For a given plate the motion should conform to rotation
about a given Euler pole. For South America (east of
the Andes), as noted above, the plate motion is consistently
to the NW with an Euler pole located at approximately
25.4°S and 126.4°W. For South America west of
the Andes the motions to the NE are consistent with
the subduction of the Nazca plate beneath the Andes.
Evidence of this phenomenon can be
seen in Figure 11 located south of the Equatorial fracture
zone in the northern South Atlantic. Here the principal
flow lines are parallel to each other but between these
flow lines the fabric of the oceanic crust suggests
a northward migration, with time, of rift axis volcanic
centres, starting close to the southern flowline and
moving progressively northwards along the rift axis,
such that the volcanic centre is now close to the northern
flowline. Since the oceanic crust is being generated
at the mid-ocean ridge, the trace of the volcanic centres
forms an inverted ‘V’ line symmetric about
the oceanic ridge (shown by yellow lines in the lower
diagram of Figure 11). This could imply that the magmatism
is more associated with the absolute motion of the plate
as discussed above. Improved satellite gravity resolution
will help to define such phenomena better and to identify
more of them. Knowing the opening rate of the MAR allows
the relative and absolute motions to be determined.
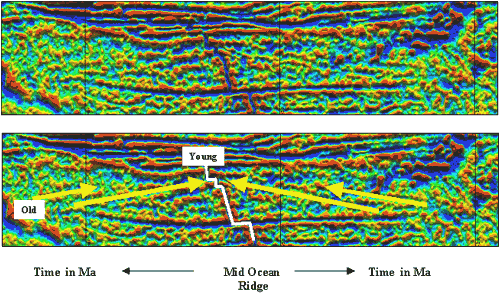
Figure 11: Structure of the mid-Atlantic
ridge based on satellite-derived free air gravity showing
migration of features (volcanic centres?) northwards
with time within a ridge segment. |
The origin of the
volcanic lineaments needs to be reassessed now that
the absolute motions of the plates are known (Figure
9). The absolute direction of plate motions is considered
strongly to influence fault tectonics (within both continents
and oceans) at both micro- and macro-scales. Faults
parallel to the direction of plate motion should be
more susceptible to reactivation, whilst faults orthogonal
to the direction of plate motion should remain locked.
On a macro-scale this may have important implications
for the early emplacement of Cretaceous kimberlites
and other centres of alkaline magmatic activity within
South America and Africa, and for the origin of the
volcanic lineaments that closely parallel the absolute
plate motion directions. On the micro-scale this same
model concept may be a principal control on the timing
of fluid flow along faults within hydrocarbon systems,
i.e. faults paralleling the plate motion being more
susceptible to fluid flow than orthogonal faults.
It is proposed that the concept of
“hotspot traces” for the South Atlantic
is over simplistic and that a model involving the periodic
release of plate stresses in the form of shear/wrench
faulting may be more appropriate. Closer evaluation
of the Walvis ridge and Rio Grande rise tends to support
such a model. The development of the Central African
rift system already testifies that major changes in
plate stress configurations, seen in the fabric of the
oceanic crust, have been able to develop and propagate
extensive passive rift systems throughout west, central
and north Africa (Figure 7). Lesser stress changes also
register within the stratigraphic record of these basins.
It is not unreasonable to conclude that stress changes
within the African and South American plates will be
absorbed or dissipated wherever there is an existing
plate/crustal weakness; the style of deformation will
depend on the orientation of such weak zones. Inspection
of the satellite gravity for the South Atlantic indicates
that deformation of the oceanic crust appears to be
quite common and may be the controlling factor in the
development of the Walvis Ridge and Rio Grande Rise
aseismic ridges. The evolutionary model that we propose
for these ridges is a periodic response to periodic
changes and release of intra-oceanic plate stress. This
has resulted in wrench and shear movement and deformation
along these features (Figure 12).
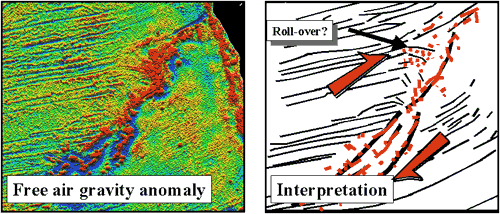
Figure 12: Free air gravity field
of the Walvis Ridge and possible interpretation in terms
of stress release at discreet times resulting in shear
movement and deformation. |
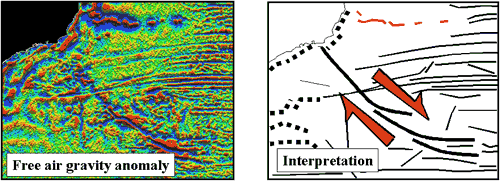
Figure 13: Image enhanced free
air gravity anomaly with possible interpretation of
dextral shear and extension in discrete segments along
the Rio Grande rise.
We propose that stress within the African plate has
been dissipated by wrench and shear movement as shown
in Figure 12 at key times in the past. The stress
release has emanated from the ridge axis and propagated
into the African plate as shear and wrench displacement,
as well as causing localised decompression melting
of the mantle, resulting in short volcanic lineaments
where the volcanic centres have the same age and terminate
at the mid-ocean ridge of the same age. The formation
of the volcanic lineament is thus not a continuous
process but is repeatedly reactivated when the plate
stresses become too great to be sustained. The previous
event will have already weakened the lithosphere such
that ensuing events reoccur along the same feature.
This then provides a model for the Walvis Ridge of
segments (illustrated as thick black lines in Figure
12) of volcanic activity progressively occurring along
with the evolution of the oceanic crust of the South
Atlantic Ocean. This model still predicts an age progression
along the ridge, but this is not necessarily linear
with distance as with the hotspot model. Evidence
for deformation for the Walvis Ridge is strong in
that there is:
-
flow line deformation, identified
as a ‘roll-over’ structure in Figure
12, suggesting dextral shear/wrench movement along
the Walvis Ridge, and
-
the orientation of the flowlines
to the north and south of the Walvis Ridge are measurably
different, consistent with such dextral motion.
A similar tectonic scheme could apply to the Rio
Grande Rise (Figure 13). Its differing bathymetric/gravity
morphology to the Walvis Ridge however suggests there
has been a greater degree of extension across the
segments.
The continental extension of the
Rio Grande trend is associated with alkaline volcanic
complexes and kimberlite diatremes which are younger
than the main phase of ca 135 Ma flood basalt volcanism
(Parana-Etendeka provinces) that pre-dates continental
break-up. Such a correlation may indicate that the
same model process as identified for the Walvis Ridge
and Rio Grande Rise is occurring deep within the continents
at the onset of the lithosphere disruption process.
If the internal structure of the Walvis and Rio Grande
ridges can be resolved better, we may gain fundamental
new insights into the origin of these intra-oceanic
plate structures and adjacent continental weak zones,
leading to the development of generic models for other
regions of the Earth.
|
5.
Conclusions
The proposed models for the volcanic
evolution of the South Atlantic could be considered
to be radically different to the model proposed by ‘hotspot’
enthusiasts. The advantage of the model is that it can
be tested in many ways since there are clear implications
for the migration of volcanism along the axis of the
MAR and for the deformation model for the formation
of volcanic lineaments. The model has the potential
to resolve the complexity of the evolving oceanic crust
since the gravity field is able to image accurately
the main tectonic events that have occurred within the
oceanic crust; these can be related to the more sensitive
indicator of sedimentary stratigraphy and associated
deformation within the adjacent continental areas of
the plate that have experienced the same changes in
plate stresses.
The overwhelming problem that is limiting
the advance of our knowledge is the resolution of the
existing satellite gravity. Our ability to repick the
altimeter radar waveforms to a higher degree of accuracy
and to improve satellite data processing methodology
using the geoid (or sea surface) to gravity rather than
the traditional method of sea slopes to gravity has
resulted in significant improvement in the data resolution
that is able to resolve reliable isotropic signals down
to 10 km wavelengths (or 5 km at half wavelength) in
equatorial areas. Such technological breakthroughs in
satellite technology still await the funds to allow
the resulting products to be used by Earth scientists
in new and exciting ways. |
Additional related information is
available from the Central Atlantic
Magmatic Province webpage and the Africa
webpage. |
- Dumoulin, C., Doin, M.-P. & Fleitout, L. (2001)
On the interpretation of linear relationships between
seafloor subsidence rate and the height of the ridge.
Geophys. J. Int. 146: 691-698.
- Fairhead, J. D. and Binks, R.M. (1991) Differential
opening of theCentral and South Atlantic Oceans and
the opening of the Central African rift system. Tectonophysics,
187: 191-203.
- Fairhead J. D., Green C. M. (1989) Controls on rifting
in Africa and the regional tectonic model for the
Nigeria and east Niger rift basins In B.R. Rosendahl,
J.J. W. Rogers and N. M. Rach, African Rifting
J. Afr. Earth Sci., 8: 231-249.
- Fairhead J. D, Green C. M. and Dickson G. W. (2001a)
Oil exploration from space: fewer places to hide.
First Break 19.9: 514 -
519.
- Fairhead J. D., Green C. M and Odegard M. E. (2001b)
Satellite-derived gravity having an impact on marine
exploration. The Leading Edge, August:
873-876
- Fairhead, J.D and Guiraud, R. (2002) Mesozoic Plate
Tectonic Controls on Rift Development in North Central
Africa: a Major Cretaceous Basin System. PESGB-HGS,
September: pp 4.
- Green C. M. and Fairhead, J. D. (1998) Satellite
derived gravity: where we are and what next. SEG
The Leading Edge, Jan: 77-79
- Maus, S., Green, C. M. and Fairhead, J. D. (1998)
Improved Ocean geoid resolution from repicked ERS-1
satellite altimeter waveforms. Geophys. J. Int.
134: 243-253.
- Muller, R.D., Roest, W.R. & Royer, J.-Y. (1998)
Asymmetric sea-floor spreading caused by ridge-plume
interactions. Nature, 396:
455-459.
- O’Connor, J.M., Stoffers, P., van den Bogaard,
P. & McWilliams. M. (1999) First seamount age
evidence for significantly slower African plate motion
since 19 to 30 Ma. Earth Planet. Sci. Lett.
171: 575-589.
- Silveira, G. & Stutzmann, E. (2002) Anisotropic
tomography of the Atlantic Ocean. Physics of the
Earth & Planetary Interiors, 132:
237-248.
- Sykes, L.R., Intraplate seismicity, reactivation
of preexisting zones of weakness, alkaline magmatism
and other tectonism post-dating continental fragmentation,
Rev. Geophys. Space Physics, 16,
621-688, 1978.
- Tucholke, B.E. & Lin, A. (1994) A geological
model for the structure of ridge segments in slow
spreading ocean crust. J. Geophys. Res.,
99: 11,958-11,978.
- Wilson, M. (1992) Magmatism and continental rifting
during the opening of the South Atlantic Ocean - a
consequence of Lower Cretaceous super-plume activity?
In: Magmatism and Continental Break-up. Special
Publication of the Geological Society 68, pp 241-255,
London.
- Wilson, M. (1997) Thermal evolution of the Central
Atlantic passive margins: continental break-up above
a Mesozoic super-plume. J. Geol. Soc. London,
154: 491-495.
|
last updated 9th August, 2004
|
|
|