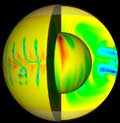 |
Global
warming of the mantle at the origin of flood
basalts over supercontinents |
N. Coltice1, B.R. Phillips2,
H. Bertrand3, Y. Ricard3 & P.
Rey4
1Laboratoire
de Sciences de la Terre, UMR-CNRS 5570, Université de Lyon,
Université Lyon 1,
ENS Lyon, Bat G’eode, 2 rue Raphael Dubois, 69622
Villeurbanne Cedex, France; coltice@univ-lyon1.fr
2Earth
and Environnemental Sciences Division, Los Alamos
National Laboratory, Los Alamos, NM 87545, USA; benp@lanl.gov
3Laboratoire
de Sciences de la Terre, UMR-CNRS 5570, Université de
Lyon, Université Lyon 1,
ENS Lyon, Bat G’eode, 2 rue Raphael Dubois,
69622 Villeurbanne Cedex, France; herve.bertrand@ens-lyon.fr ; ricard@ens-lyon.fr
4School of Geosciences,
University of Sydney, H11 Geology Demountables, NSW2006
Sydney, Australia
Click here to
download a PDF version of this webpage
Click here to go to
discussion of this page
Introduction
In a recent paper (Coltice et
al.,
2007), we
proposed an alternative, non-plume model for the generation
of continental flood basalts (CFBs) over a supercontinent.
A supercontinent imposes its length-scale to the convective
flow which becomes less efficient to remove heat. Thus,
the subcontinental mantle heats up by around 100°C
triggering large-scale melting. This hypothesis is
based on the peculiarities of the Central Atlantic
Magmatic Province (CAMP) which is the largest phanerozoïc
CFB on Earth (~106 km2), and
3D spherical models of mantle convection with continents
[Ed: see also CAMP page].
The CAMP emplaced
at a peak rate at 199 Ma during
the initial breakup of Pangea and is now preserved
over four continents. It is often
cited as a type example of a plume-derived CFB
(Hill, 1991; Courtillot
et al., 1999) but this hypothesis
is strongly debated because:
-
-
the geometry
of the CAMP is elongated, not radial as would be
expected from a plume,
-
the area near
the center of the hypothetic plume head does not show
evidence of uplift ( McBride, 1991; McHone,
2000),
-
the apparent radiating pattern of the feeder
dyke swarms that would result from the impingement
a plume head is an oversimplification ignoring the
regional lithospheric control (McHone
et al.,
2005),
-
the geochemical and isotopic
signatures are diagnostic of shallow mantle sources
that experienced ancient subduction and do not
have a deep plume composition (Verati et al.,
2005).
In
this webpage we show how continental aggregation favors
longer lengthscales of flow which naturally generates
subcontinental warming of 100°C without
the involvement of hot active plumes. Our model supports
and quantifies the idea of Anderson (1982) who proposed that continental assembly would cause
an increase in mantle temperature and the breakup of
Pangea.
The global mantle warming hypothesis
Plumes carry the heat coming from
the core and heat up the lithosphere locally. Without
plumes, it is difficult to have significant temperature
oscillations on a 100-Ma timescale unless there is
a drastic change in the convective flow pattern. Our
hypothesis is that continental aggregation generates
a longer wavelength of convection so that the subcontinental
mantle can heat up sufficiently to generate melting
over a large area. Indeed,
it is well known that:
- longer wavelengths are less
efficient at removing heat (Grigné et
al., 2005), and
- continental rafts impose
their own wavelength on mantle convection (Phillips
& Bunge,
2005) by impeding downwellings
below them (Gurnis, 1988).
As a consequence,
the assembly of a supercontinent should force
larger lengthscales and drive the underlying
mantle toward higher temperatures, even in the
absence of plumes.
Model testing
To test this hypothesis, we set up
numerical models of mantle convection incorporating
continental lithosphere. The models are purely heated
from within in order to eliminate hot plumes. We refer
to our paper (Coltice
et al.,
2007) for details of the 2D cartesian
and 3D spherical models. The first set of experiments
aims at characterizing the role of continental distribution.
Thus the models have stationary continents. They show
that the temperature below a supercontinent is ~100°C
hotter than with 2 separate continents (Figure 1),
regardless of the geometry (2D cartesian or 3D spherical)
or the technique used to model the continental lithosphere.
With moving continents, the temperature is stable until
aggregation starts and then it takes more than 100
Ma to heat up the subcontinental mantle by 100°C
(Figure 2).
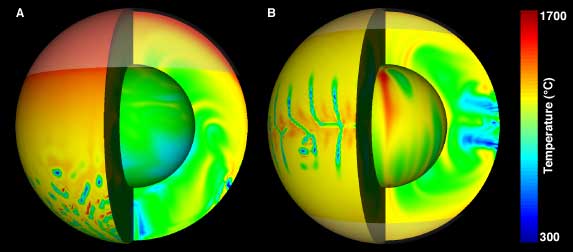
Figure 1. Temperature field snapshots
for models with (A) a supercontinent and (B) two
antipodal continents. The mean temperature at the
base of the continental thermal boundary layer in
(A) is 1614°C (red),
while in (B) it is only 1475°C (yellow). Translucent
caps denote continents. The outer surface
is at 100 km depth. Heating is purely internal with
a heat production rate of H = 4 x 10-12 Wkg-1,
viscosity is layered, and the Rayleigh number Ra =
107. The linear features on the planetary
surfaces delineate regions of cold, subducting material.
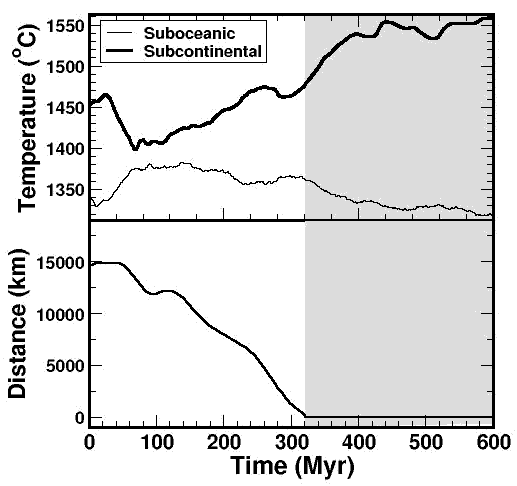
Figure 2. Temporal evolution
of the distance between two moving continents and
average temperatures in an internally heated 2D convection
simulation at Rayleigh number Ra = 108.
Time is scaled by the transit time (30 Ma) which
is the time it takes to cross the mantle at the
surface horizontal velocity (Gurnis, 1988). The heat
production is H = 2.6 x 10-12 Wkg-1.
Plumes vs. global mantle warming
below a supercontinent
We suggest that reorganization
of the flow during continental aggregation can be responsible
for a positive temperature excursion up to 100°C,
which might be an upper bound considering
some of the shortcomings of our models. Such a large-scale
thermal anomaly would be sufficient to partially melt
the subcontinental mantle (Anderson,
1982),
especially if the lithospheric mantle is hydrated,
since the edges of colliding continents are vanished
subduction zones. The temperature anomaly generated
by the global mantle warming is wide and diffuse. It
dissipates with continental dispersal and would not
leave a hotspot track on the seafloor. Magma drainage
is controlled by the lithospheric and tectonic setting.
Of course, our model is an end-member and does not
preclude plumes. A combination of the two might occur
and should be investigated with convection models.
Thus, we propose two end-member mechanisms
for CFB generation:
Global
mantle warming
-
Wide and
diffuse magmatism (e.g CAMP: 7000
km)
-
No hotspot
track
-
< 100°C excess
temperature
-
Low rate
of magma supply
-
Shallow mantle source
-
A supercontinent is needed
|
Plume
- Radiating magmatism
over a restricted area (< 2000 km)
- Linked with
a hotspot track
- 200°C excess temperature
- High rate of
magma supply
- Deep mantle source
- Anywhere
|
The global mantle warming model accounts
for the characteristics of the CAMP and might also
apply to other CFBs such as the Karoo [Ed: see also Karoo page].
The CFB linked to the breakup of the supercontinent
Pannotia during late Neoproterozoic times (the Central
Iapetus Magmatic Province (CIMP); Doblas
et al., 2002) might also be due to global mantle
warming. This model also offers an alternative view
to explain episodic creation of juvenile crust from
the upper-mantle (Condie, 2004) without invoking
deep-seated mantle plumes.
Acknowledgments
We thank Gillian Foulger for inviting
this contribution.
References
-
-
-
Condie, K.C., Supercontinents
and superplume events: distinguishing signals in
the geologic record, Physics
of the Earth and Planetary Interiors 146, 319–332,
2004.
-
Courtillot, V., Jaupart, C.,
Manighetti, I., Tapponnier, P., and Besse, J.,
On causal links between flood basalts and continental
breakup, Earth and Planetary
Science Letters 166,
177–195, 1999.
-
Doblas, M., Lopez-Ruiz,
J., Cebria, J.M., Youbi N., and Degroote E., Mantle
insulation beneath the west African craton during
the Precambrian-Cambrian transition, Geology, 30,
839-842, 2002.
-
Grigné, C., Labrosse,
S., and Tackley, P.J., Convective heat transfer
as a function of wavelength. Implications for the
cooling of the Earth, Journal
of Geophysical Research 110, B03409, 2005.
-
Gurnis, M.,
Large-scale mantle convection and the aggregation
and dispersal of supercontinents, Nature 332, 695–699,
1988.
-
Hill, R.I., Starting plumes
and continental break-up, Earth
and Planetary Science Letters 104, 398–416,
1991.
-
McBride, J.H., Constraints on the structure and
tectonic development of the early Mesozoic south
Georgia rift, southeastern United States; seismic
reflection data processing and interpretation, Tectonics 10, 1065-1083, 1991.
-
-
McHone, J.G., Anderson, D.L.,
Beutel, E.K., and Fialko, Y.A., Giant dykes, flood
basalts, and plate tectonics: a contention of mantle
models, Geological Society
America Special Paper 388,
p. 401, 2005.
-
-
Verati, C., Bertrand, H., and
Féraud, G.,
The farthest record of the Central Atlantic Magmatic
Province into West Africa craton: precise 40Ar/39Ar
dating and geochemistry of Taoudenni basin intrusives
(northern Mali), Earth and
Planetary Science Letters 235, 391–407,
2005.
Discussion
6th August, 2007, Sami Mikhail (s.mikhail@gl.rhul.ac.uk)
I
recommend that all who found the modelling of this
webpage and Coltice
et al. (2007) interesting
read also Yale & Carpenter (1998). This
previously published work supports it in many
ways. The authors show a temporal link between
supercontinent assembly and break-up with LIP emplacement.
This suggests that the supercontinents cause a reduction
in heat flow out of the mantle simply by insulating
it. This can give rise to thermal anomalies, or ‘hot
spots’ in the upper mantle, and generate the
heat for continental flood basalt (CFB) petrogenesis.
So either
theses regions heated up enough to melt and form
giant dyke swarms and possibly continental large igneous
provinces (LIPs), and drive rifting, or supercontinent
rifting caused by plate tectonic processes caused these
heated regions to decompress thus triggering CFBs.
This model
is useful for explaining CFBs
but not ocean island basalts. It requires no
deep-mantle-sourced thermal anomaly (plume), just a
progressive build-up of heat caused by lithospheric
insulation of the mantle by (super)continents, i.e., top
down tectonics (Anderson,
2001).
The global warming model (Yale
& Carpenter,
1998;
Coltice
et al.,
2007) can be used to explain some CFBs
(Siberia: Yale
& Carpenter,
1998; CAMP: Coltice
et al.,
2007) using observation and modelling. However
it falls short in explaining the mechanisms responsible
for the petrogenesis of oceanic plateaus (e.g.,
the Ontong
Java plateau) and ocean island chains (e.g., the
Emperor
seamount chain).
Coltice
et al.,
2007 compare their model to the plume model,
but I have a few problems:
- Why must we assume that
plumes are shaped like tadpoles causing radial
magmatism with a predictable radius? Surely the
geometry of any thermal plume will be controlled
by the laws of thermodynamics? Thus the direction
in which the energy (heat) is able to move
defines its shape?
- Why, when discussing the dynamic
Earth, must the geometry and size of a plume be
regarded as uniform?
- What about giant dyke swarms as fractals
for ‘plumes’?
- The idea that all plumes
must have a hotspot track is primitive.
If we were to have a plume that has a replenishing
source beneath a moving plate then yes it should
have a hotspot track. However if the plume is caused
by an instability that is short lived it may not
produce a hotspot track, but just a single LIP.
What is
wrong with a plume only producing a single LIP
and then dying out?
If one can devise a model that can
explain the petrogenesis of a specific LIP (e.g.,
Coltice et
al., 2007 with
CAMP), then it explains the petrogenesis of the LIP
in question, not all LIPs. In a similar way, one could
argue that volcanoes are found in many geological settings,
including subduction zones, rift zones and my favourites
the tectonically inert ‘hot-spots’.
We would be wrong, however, to study Santorini and
conclude that all volcanoes on Earth are a direct result
of subduction. Surely the same goes for LIPs.
8th August, 2007, Don Anderson (dla@gps.caltech.edu)
Mikhail raises valid points about
the philosophy of science and the process of falsification
and asks a series of astute questions. These issues
are discussed
elsewhere.
The geometry of a thermal plume is indeed controlled
by the laws of thermodynamics. This means that not
only must the effects of temperature be considered
(for density, elastic moduli, viscosity, expansivity,
conductivity, specific heat) as in plume theory, but
also the effects of pressure. The Earth is too big
to ignore pressure and thermodynamics requires that
volume changes are associated with both heating
and compression.
Pressure reverses the effects of temperature and broadens
plumes with depth, if they can form at all. Narrow
plumes do not exist when self-consistent thermodynamics
is allowed for. Internal heating leads to broad diffuse
upwellings, that move around so that heat can be removed
from all parts of the interior. Deep slabs cool off
the mantle from below. These are the opposite of the
plume scenerio.
Continental delamination is an instability that is
short lived and may not produce a hotspot track, just
a single LIP at the delamination site, and another
one when the fertile blob emerges. Many mechanisms have been proposed that produce a single LIP and then
die out.
The process by which large thick plates trap mantle
heat is usually referred to as continental
insulation. This process also applies to any
large long-lived plate, e.g., Pacific (Parmentier
& Sotin, 2000) or plates in compression. More
generally, the presence of a lid that is either buoyant
or has strong
subduction zones can cause the whole mantle
to run a fever compared to a homogeneous fluid with
a purely thermal boundary layer (TBL) that becomes
unstable when still relatively thin. Mantle temperature
in this case is not buffered by mantle viscosity.
A realistic mantle model not only has a variable thickness
crust, lithosphere and TBL, but also has the
accumulated refractory depleted buoyant peridotite
debris of billions of years of melt extraction, the
perisphere. When the crust thickens into the eclogite
stability field, near 50 km depth, it can delaminate
and place mafic low-melting components into the upper
mantle, thereby cooling it. Such a mantle operates
differently from a homogeneous fluid heated from below,
e.g., the plume scenerio. If the mantle is only slightly
hotter than generally assumed then plumes are both
unnecessary and implausible. But it is homologous
temperature, rather than absolute temperature,
that is the key parameter.
The insulation model is useful for explaining
both CFBs and ocean island basalts (Anderson,
2000, 2001) and the high ambient temperature of the
mantle. A more fundamental issue is the following;
what is the ambient temperature of a mantle, insulated
from above, that is unaffected by deep mantle
plumes? Can it be as high and as variable as 1420 ± 180°C
(Kaula, 1983; Green et
al., 1999; temperature
pages)? Related
questions are;
- Can we assume that the mantle is subsolidus
except at plate boundaries and at hotspots?
- Are mid-ocean
ridge potential temperatures and melting points
representative of the whole upper mantle,
including under large plates?
- Can large plates or strong
subduction zones cause the mantle to overheat?
- Does lower continental crust delamination cool
the mantle under supercontinents, so that it is actually
colder than under large oceanic plates?
- Why are there
not volcanoes everywhere?
Note: phrases in boldface are Googlets.
Enter these into a search engine for supplementary
material.
9th August, 2007, Nicolas Coltice
(coltice@univ-lyon1.fr)
& Benjamin R. Phillips
There are many proposed hypotheses for the origin
of LIPs and it was not the objective of our work
to propose another. As we stated, the role of a supercontinent
has already been highlighted by Anderson (1982). Our
goal was then to test the hypothesis with dynamic simulations.
Indeed, there is a difference between a hypothesis
and a model. A model can be used to make predictions
that can be compared to independent datasets. Contrary
to Yale & Carpenter (1998), we propose
simulations and make predictions e.g., the
temperature below a continent as a function of its
size, the shape of the thermal anomaly, and the time
needed to increase the temperature. All of these
can be tested independently now. Second, we explained
the physics associated with the phenomenon. As a consequence,
we can predict that each time there is a supercontinent,
~100°C of
subcontinental warming occurs. Our model is not designed
to explain the CAMP but to show a general physical
mechanism.
This mechanism is related to continental
insulation. But if there is no convection simulation
to explore what continental insulation really is, many
questions could be raised such as:
- Is the thermal effect of continental insulation
a function of the total area of continents and/or
of the distribution of continents?
- Does the size of a continent affect its sublithospheric
temperature?
Most numerical models that study the impact of continents
on the mantle explore the effects of basal heating
and plumes, very often below a single continent (Gurnis,
1988; Lowman & Jarvis, 1999; Lenardic
et al., 2003).
We investigated the effects of the distribution of
continents on mantle convection heated from within
and were able to make a link with a proposed hypothesis
(Anderson, 1982) and a peculiar CFB for which
a huge amount of data is available (the CAMP).
Concerning the size and shape of plumes, we totally
agree that it is not as simple as a little mushroom,
especially taking into account chemical anomalies at
the base of the mantle (Farnetani
& Samuel, 2005).
However, plumes are small because their viscosity is
low and this is why tomographic models fail to see
some of them. The tail developed by plumes must exist
at least for some time. Indeed, a rising viscous drop
experiences stresses (pressure gradients and viscous
stresses) on its boundary and the velocity gradients
produce the tail that sometimes can be short-lived.
The shape of the CAMP is not the only observation
that leads us to question the plume model and more
is discussed elsewhere by McHone (2000) [Ed:
and in the CAMP webpage]. It
is difficult to build a testable hypothesis, test it
against the law of physics, make predictions and find
the unambiguous independent observations. But one of
the roles of modellers is to make quantitative predictions
that distinguish a realistic model from a reasonable
hypothesis.
Discussion references
- Anderson, D.L., The thermal state of the upper
mantle; no role for mantle plumes, Geophys.
Res. Lett., 27,
3623–3626,
2000.
- Anderson, D.
L., Top-down tectonics?, Science, 293, 2016-2018,
2001
- Coltice,
N., B.R. Phillips, H. Bertrand, Y. Ricard, P. Rey,
Global warming of the mantle at the origin of flood
basalts over supercontinents, Geology 35,
391-394, 2007
- Courtillot, V., Davaille, A., Besse,
J., Stock, J., Three distinct types of hotspots
in the Earth's mantle, Earth and
Planetary Science Letters, 205,
295–308, 2003.
- Farnetani, C.G., H. Samuel, Beyond the thermal
plume paradigm, Geophys. Res. Lett., 32,
L07311, 2005.
- Green, D. H., T. J. Falloon, S.M. Eggins
and G.M. Yaxley, Primary magmas and mantle
temperatures, European
J. Min., 13, 437-451, 1999.
- Kaula, W.M., Minimum upper mantle temperature
variations consistent with observed heat flow and
plate velocities, Journal of Geophysical
Research, 88, 10323–10332,
1983.
- Kelley, K.A., T. Plank, S. Newman, E.
Stolper, T.L. Grove, and S. Parman, Mantle melting
as a function of water content in arcs, Eos
Trans. AGU, 84 (46), V41D-06,
2003.
- Lenardic, A., L.-N. Moresi, H. Mühlhaus,
Longevity and stability of cratonic lithosphere:
Insights from numerical simulations of coupled
mantle convection and continental tectonics, J.
Geophys. Res., 108, DOI
10.1029/2002JB001859, 2003.
- Lowman, J.P., G.T. Jarvis, Continental
collisions in wide aspect ratio and high Rayleigh
number two-dimensional mantle convection models, J.
Geophys. Res., 101, 25485-25498,
1996.
- McHone, J. G., Non-plume magmatism and tectonics
during the opening of the central Atlantic Ocean, Tectonophys., 316,
287-296, 2000.
- Parmentier, E.M. and Sotin, C., Three-dimensional
numerical experiments on thermal convection in a
very viscous fluid: Implications for the dynamics
of a thermal boundary layer at high Rayleigh number, Physics
of Fluids, 12, 609-617, 2000.
- Sen,
G., S. Keshav and M. Bizimis, Hawaiian mantle xenoliths
and magmas: Composition and thermal character of
the lithosphere, Am.
Mineral. 90, 871-887,
2005.
- Yale,
L.B., Carpenter, S.J., Large igneous provinces
and giant dike swarms: proxies for supercontinent
cyclicity and mantle convection, Earth and
Planetary Science Letters, 163,
109–122, 1998.
last updated 9th
August, 2007 |