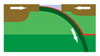 |
Origin
of the Iceland hotspot and the North Atlantic Igneous
Province |
Jun
Korenaga
Department
of Geology and Geophysics, Yale University, P.O. Box
208109, New Haven, CT 06520-8109
jun.korenaga@yale.edu
Click here to
download a PDF version of this webpage
Introduction
To understand the origin of the Iceland
hotspot, we must look at the big picture and start from
the continental-breakup magmatism that took place during
the opening of the North Atlantic around 60 million
years ago. Because of spatial and temporal continuity
in anomalous magmatic activity, underlying mechanisms
for the Iceland hotspot and the North Atlantic igneous
province must be closely connected, if not the same.
We may also look at an even bigger
picture (Figure 1). The North Atlantic is not the only
place where massive breakup magmatism occurred. Large
igneous provinces on a similar scale were also created
during the opening of the Central Atlantic (the US East
Coast igneous province formed at ~200 Ma) as well as
the South Atlantic (the Paraná and Etendeka flood
basalt provinces formed at ~120 Ma) (Editor's note:
see also the Central Atlantic Magmatic
Province and South Atlantic
pages).
Figure 1.
Most Atlantic large igneous provinces are related
to the dispersal of the supercontinent Pangea,
which initiated ~200 million years ago. Red areas
denote the distribution of large igneous provinces
that have been recognized so far, and yellow circles
denote currently active hotspots (after Coffin
& Eldholm [1994] and Schilling [1985]).
|
This rather frequent formation of
large igneous provinces during the disintegration of
the supercontinent Pangea is a surface manifestation
of the thermal and chemical state of convecting mantle
beneath the supercontinent. Something unusual (with
respect to normal convecting mantle) is required, otherwise
normal seafloor spreading would have taken place. The
impact of a starting mantle plume (Figure 2) has been
a popular hypothesis for the last decade or so, but
recent geochemical and geophysical findings from the
North Atlantic igneous province all point to the significant
role of incomplete mantle mixing in igneous petrogenesis.
Figure 2. The most
popular hypothesis for continental breakup magmatism
is the arrival of a mantle plume. The plume impact
hypothesis is a theory that attributes anomalous
mantle melting to thermal anomalies in convecting
mantle. For the melting of such hotter-than-normal
mantle, we can make various geophysical and geochemical
predictions, which can be compared with observations
to validate the hypothesis.
|
In this essay, I first summarize key
observational constraints on the nature of parental
mantle dynamics for continental breakup magmatism. Available
evidence appears to favor a hypothesis in which anomalous
magmatism results from fertile mantle, not from anomalously
hot mantle. However, this hypothesis has an often-neglected
dynamical issue – fertile mantle without a thermal
anomaly is denser than normal mantle and it should sink
instead of rise, as O'Hara pointed out in 1975. I will
show that this dynamical dilemma can be resolved quite
naturally if the multi-scale nature of mantle convection
is properly taken into account. Multi-scale mantle mixing
could potentially explain a variety of hotspot phenomenology
as well as the formation of both volcanic and non-volcanic
rifted margins, with a spatially- and temporally-varying
distribution of fertile mantle.
Observation
1: Mantle Tomography
Both S-wave and P-wave
global tomography models clearly show that a strong
low-velocity anomaly beneath Iceland ends sharply at
the 660-km discontinuity. It is important to note that
this shallow feature of the Iceland low velocity anomaly
is supported by both S-wave and P-wave
models. S-wave models are mainly constrained
by surface waves (which are sensitive only to upper
mantle structure) and body waves (which have good resolution
only for the lower mantle in case of Iceland). The continuity
of low-velocity structure might be influenced by how
one weights these different data sets in tomographic
inversions. The new finite-frequency P-wave
tomography models of the Princeton group (which benefit
from more resolving power for a given ray coverage)
provide strong confirmation for earlier S-wave
models for the Iceland hotspot, in this respect.
Figure 3. Global
S-wave mantle tomography by J. Ritsema and coworkers
(Model S20RTS: the so-called "Caltech model").
Cross sections beneath Iceland sliced at three
different angles are shown [from Ritsema &
Allen, 2003]. This model is derived from free-oscillation
splitting, surface-wave dispersion, and body-wave
traveltime measurements.
|
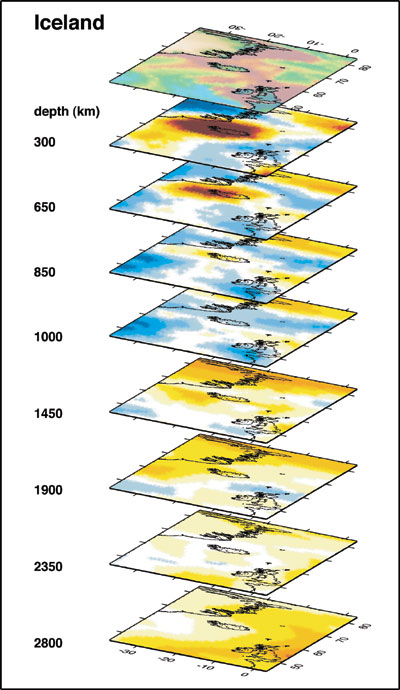
Figure
4. Global P-wave mantle tomography
by Montelli et al. [2004] (the "Princeton model"),
based on P and PP traveltimes measured at two
different frequencies. |
Observation 2: Crustal
Tomography
Melting of hot plume mantle is also
irreconcilable with the crustal structure of the Greenland
Tertiary igneous province. Hotter mantle produces a
larger amount of melt, with increasing MgO content (because
of a larger olivine component in the melt). Roughly
speaking, the seismic velocity of igneous crust is mainly
controlled by this MgO content. Higher MgO leads to
higher crustal seismic velocity. Therefore, there should
be a positive correlation between crustal volume
and crustal velocity, if thick igneous crust is produced
by melting anomalously hot mantle. This is not observed
on the Greenland margin part of the North Atlantic igneous
province (Figure 5). Clearly, something other than the
melting of hot mantle is involved. Crustal structure
alone does not tell us what this is, exactly, so we
need to consider complementary information such as mantle
geochemistry. |
Figure 5. (upper
left) Seismic transects across the
southeast Greenland margin surveyed
during the 1996 SIGMA experiment by
WHOI and DLC [Holbrook et al., 2001].
So far, only Line 2 has been modeled
by a tomographic method. Preliminary
modeling of the other three transects
by (semi-)forward modeling has been
published, pending a more complete
tomographic study with proper quantification
of model reliability. (upper right) Correlation
between P-wave velocity and thickness
as seen in the Line 2 crustal model
(blue dots with error bars; excluding
the contribution from the continental
crust component). Red line denotes
a theoretical prediction for the case
of a thermal plume (see Korenaga et
al. [2002] for details.)
(bottom) P-wave velocity model for Line
2. For technical details on the tomographic
method, see Korenaga et al. [2000].
|
Observation 3: Major Element Chemistry
Primary melt compositions estimated for the North Atlantic
igneous province suggest that (1) the source mantle
is fertile (i.e., Mg#~86-87) probably resulting from
the addition of recycled crust and (2) the source mantle
is not notably hot with respect to MORB source mantle
[Korenaga & Kelemen, 2000]. It should be
noted that estimating primary melt composition is not
an easy task. Surface lava composition is usually very
different from primary melt because of various fractionation
processes. How do we get something like Figure 6?
Korenaga & Kelemen [2000]
devised a fractionation-correction method based on liquid
Ni concentration. Ni is only a compatible trace
element for olivine, which is the most abundant phase
in the upper mantle. Thus, the Ni content in mantle
olivine is expected to be stable in the face of any
previous depletion and enrichment history. Indeed, compilation
of mantle xenoliths and abyssal peridotite demonstrates
that the Ni content clusters tightly between 2500 ppm
and 3500 ppm. We can safely use this range as a reference
when we apply back-fractionation correction to surface
lava data.
This Ni-based fractionation correction
is not a new idea. It had already been proposed, for
example, by Allegre et al. [1977]. However,
we now have much better control on Ni partitioning between
olivine and basaltic melt from a large number of laboratory
data, and also the distribution of Ni content in mantle
olivine is better understood. Furthermore, the correction
method is only applicable to relatively primitive samples
(with MgO content greater than ~9 wt%). The North Atlantic
igneous province is, fortunately, one of the best studied
areas in terms of petrology, and the number of suitable
samples is sufficiently large.
To test if this revived estimation
method really works, Korenaga & Kelemen
[2000] first applied it to normal mid-ocean ridges.
Estimated primary compositions all cluster around the
composition space in equilibrium with source mantle
of Mg#~0.89, which is a pyrolitic value (see yellow
patches in Figure 6). Furthermore, the Indian Ridge,
Northern EPR, and the Kolbeinsey Ridge extend most of
the “global trend” of mid-ocean ridge basalt
composition [Klein & Langmuir, 1987]. As
shown in Figure 6, inferred melt temperature increases
from the Indian Ridge (including the Australia-Antarctica
Discordance) to the Kolbeinsey Ridge (referred to as
“Iceland” in the complilation of Klein
& Langmuir [1987]), consistent with expected
temperature variations along the mid-ocean ridge system.
Figure 6.
Estimated primary melt compositions in terms of
MgO and FeO for the North Atlantic igneous province
(East Greenland, Southeast Greenland, Southwest
Iceland, Theistareykir (North Iceland), Reykjanes
Ridge, and Kolbeinsey Ridge) and normal mid-ocean
ridges (Northern EPR, North Atlantic, and Indian
Ridge). Also shown are the contours of melt temperature
(dotted) and corresponding Mg# of source mantle
(solid). See Figure 7 for how to interpret this
primary composition. (From Korenaga & Kelemen,
2000) |
By looking at primary melt compositions
for the North Atlantic igneous province, one notices
that, whereas the Kolbeinsey Ridge and Theistareykir
appear to be similar to normal mid-ocean ridges, other
localities exhibit a distinctly different trend with
much lower Mg#. This distribution is not random. The
Kolbeinsey Ridge is located north of Theistareykir,
and they are geographically connected. There appears
to be some kind of compositional dichotomy in the source
mantle for this igneous province. At any rate, the bulk
of the North Atlantic igneous province is characterized
by unusually low Mg#, i.e., more iron-rich source mantle,
which is more prone to melting because of its lower
solidus. In addition, the temperature of the primary
melt is not very different from normal mid-ocean ridges
(Figure 7). (Note: Do not confuse the temperature of
primary melt with the temperature of erupted lava, which
does not have a direct connection with the potential
temperature of the source mantle.)
Figure 7. Primary
melt composition in MgO-FeO space tells us two
things. (1) Mg# constrains the fertility of
the source mantle. Any melt from pyrolite (i.e.,
normal) mantle has equilibrium olivine Mg# greater
than ~0.89. To create melt with a lower Mg#,
the source mantle composition must have lower
Mg#. (2) Mg-Fe partitioning between olivine
and basaltic melt is sensitive to temperature,
so one can convert melt composition into melt
temperature. By applying this geothermometer
to primary melts (not erupted lavas), one can
estimate how hot the source mantle was when
it was chemically in equilibrium with melt (in
an averaged sense, because primary melt is an
aggregate of polybaric melts).
|
Observation 4: Isotope
Geochemistry
The simplest way to lower the Mg# of
the source mantle is to add subducted crust to pyrolitic
mantle. Assuming that the source mantle is indeed such
a composite of recycled crust and normal mantle, we
can use isotope data to constrain the nature of the
recycled crust. Pb, Nd, and Hf isotope data suggest
that the original formation age of the recycled crust
must be relatively young, in the range of 0.5-0.7 Ga
[Hanan & Graham, 1996; Korenaga & Kelemen,
2000; Lesher, 2002] (Figure 8). Interestingly,
this estimated age is consistent with the closure of
the Iapetus (proto-Atlantic) Ocean. Based on this coincidence,
Korenaga & Kelemen [2000] speculated that
the North Atlantic igneous province might originate
in the short-term recycling of the Iapetus MORB. This
short-term recycling is most compatible with upper-mantle
dynamics.
It has been suggested that Pangea
experienced significant northward motion since the Caledonian
orogeny and that this motion destroyed a direct link
between subducted crust and the suture. It should be
noted, however, that it is impossible to resolve the
relative motion of a supercontinent with respect
to the underlying mantle from paleomagnetic data because
of the possibility of true polar wander. The geology
of Pangea's leading edge suggests that true polar wander
most likely occurred during the Permo-Triassic [Marcano
et al., 1999], in which case the entire mantle
rotated as a whole and no significant shear is expected
between Pangea and sublithospheric mantle. Thus, the
northward migration of the supercontinent does not pose
an impediment to short-term recycling.
|
Figure
8. Covariation of epsilon Nd and
estimated whole rock Mg# for the six regions in
the North Atlantic igneous province. To illustrate
the effect of basalt addition, mixing curves between
depleted pyrolite mantle and 0.5 Ga (solid) or
0.7 Ga (dotted), normal MORB are also shown. See
Korenaga & Kelemen [2000] for details.
|
Dynamical Dilemma
To lower the Mg# of the source mantle
to as low as ~0.87, we need to add a very large amount
of recycled oceanic crust (Figure 9); the volume fraction
of the recycled crust should be as much as 20-30% of
the source mantle. Whereas such fertile mantle can result
in a very high-degree of melting without a notable thermal
anomaly, it is much denser than normal pyrolite. Such
fertile mantle requires an excess temperature anomaly
of 200-300K to become just neutrally buoyant. How can
such dense mantle rise in the first place, without a
large thermal anomaly? Because fertile mantle has an
intrinsic density excess, its potential contribution
to excess magmatism has often been discounted at the
outset - it appears to be simply physically implausible.
Previous numerical studies on the dynamics of fertile
mantle (e.g., eclogite-bearing plumes) [Cordery
et al., 1997; Leitch & Davies, 2001]
have circumvented this density issue by neglecting it
(!), which does not seem to be an attractive solution.
As explained below, however, the upwelling of dense
fertile mantle may be a natural consequence of multi-scale
mantle dynamics.
|
|
Figure
9. (left) Mg# of source mantle
can be affected by addition of recycle oceanic
crust. The influence of this addition on whole-rock
Mg# is not linear, and to lower Mg# down to ~0.87,
as 30% of recycled oceanic crust is required to
be mixed with pyrolitic mantle. (right)
Because basalt is transformed into eclogite at
a depth of ~50-60 km, basalt addition to normal
mantle results in an increase in bulk density
(after Ringwood & Irifune [1988]). The density
difference of 0.1 g/cm3 is equivalent
to a temperature anomaly of 1000 K.
|
Solution: Whole-mantle Sublithospheric
Convection
Sublithospheric convection driven by
surface cooling can naturally resolve the above dynamical
dilemma, when considered in the whole-mantle framework.
Sublithospheric convection, which is often called small-scale
convection, has been traditionally studied in an upper-mantle
framework. The base of the upper mantle (i.e., the 660-km
discontinuity) is not necessarily an impermeable boundary.
For a relatively short duration (e.g., ~100
Myr), it can be considered as impermeable, but realistic
viscous layering and the endothermic phase change expected
at this depth is not strong enough to maintain the layered
convection state forever. Such a layered state eventually
breaks down, and when this happens, strong counter upwelling
from the lower mantle is expected to compensate downwelling
from the upper mantle. This mass-conserving flow, which
takes place together with buoyancy-driven flow, could
be strong enough to entrain dense fertile mantle and
bring it to the surface.
|
Figure 10. Concept
of whole-mantle-scale sublithospheric convection
and mass-conserving counter upwelling. This rich
multi-scale dynamics of sublithospheric convection
was not recognized until very recently.
|
New Working Hypothesis
Voluminous breakup magmatism may
simply reflect compositional heterogeneity in the upper
mantle, not excess mantle temperature associated with
a deep thermal boundary layer. With the above counter-upwelling
mechanism, we are now ready to build a new working hypothesis
for the origin of the North Atlantic igneous province
as well as the Iceland hotspot. To make a hypothesis
that can satisfy all the major observational constraints
is difficult. So far I have been able to come up with
only one dynamically-consistent scenario, which is shown
below. I am not aware of any alternative hypotheses
that have a similar capability.
|
Figure 11.
Schematic illustration of a possible scenario
for the formation of a large igneous province
during the breakup of Pangea. (a) The closure
of the Iapetus Ocean took place prior to ~500
Ma, and during this period, a layer of oceanic
crust was segregated from the subducting slab
at the base of the mantle transition zone. (b)
The Iapetus Ocean diminished, and the Caledonian
Orogeny took place during ~500-400 Ma. (c) The
supercontinent Pangea was assembled during ~320-170
Ma, and sublithospheric convection was initiated
beneath suture zones. Though convective motion
is depicted here two-dimensionally, it is most
likely three-dimensional, with strong convection
confined in the out-of-plane direction. (d) Sublithospheric
convection was confined in the upper mantle for
a while, but eventually this layering broke down,
and mass-conserving counter upwelling entrained
crustal components to the base of lithosphere.
(e) Plate-driven flow during continental rifting
(e.g., ~60 Ma for the North Atlantic) further
assisted this entrainment process, and the circulation
of fertile mantle to shallow depths resulted in
a high degree of melting, which is observed as
flood basalt volcanism. |
The crustal component of a subducting
slab has a higher viscosity than the lithospheric component
and ambient mantle because of its higher garnet content,
and it also becomes strongly buoyant below the 660-km
discontinuity. Segregation of oceanic crust at the base
of the mantle transition zone is debatable, but at least
it is physically plausible if these viscosity and density
contrasts are taken into account [e.g., Karato,
1997]. This segregation stage (Figure 11a,b) is essential
for creating fertile mantle. If subducted crust and
depleted lithosphere is simply remixed the original
pyrolite mantle only is recovered, which is not anomalously
fertile.
The initiation of sublithospheric
convection (Figure 11c) is a natural consequence of
surface cooling, which takes place continually everywhere
on Earth. This convection mechanism is fundamentally
different from so-called “edge-driven” convection,
which requires a special (and probably unrealistic)
setup of lithospheric structure and asthenospheric condition
(see Korenaga & Jordan [2001, 2002] for
detailed discussion. Editor's note: see also EDGE
page).
Mass-conserving counter flow and any
entrainment of oceanic crust (Figure 11d) does not necessarily
take place beneath the suture zone as depicted in the
cartoon. Counter flow could take place somewhere else;
if it happens beneath a thick continental craton, it
would not be manifest in the surface geology. Also,
even if counter flow does occur beneath a suture zone,
entrainment would not take place without crustal fragments
floating at 660 km. This may explain the limited occurrence
(both spatially and temporally) of flood basalt provinces
during the opening of the Atlantic.
Once raised to shallow depths (Figure
11e), crustal components start to melt much earlier
than surrounding mantle. The liquidus of the crustal
component is lower than the solidus of the surrounding
pyrolite; adiabatically upwelling mantle with a potential
temperature of 1623 K, for example, intersects the solidus
and liquidus of anhydrous mid-ocean ridge basalt at
~150 km and ~70 km, respectively [e.g., Yasuda
et al., 1994]. This preferential melting of crustal
fragments results in strong melt-retention buoyancy,
which can further enhance upwelling.
Counter-flow entrainment can bring
up fertile mantle to very shallow depths. This is important,
because it can explain the formation of a flood basalt
province as soon as continents are rifted apart. If
plate-driven flow is the only mechanism that drags up
dense crustal fragments, temporal coincidence between
rifting and anomalous magmatism cannot be explained.
Numerical Modeling of Counter-Flow
Entrainment
To test the feasibility of this new
working hypothesis, especially the counter-flow entrainment
scenario, some preliminary numerical modeling has been
done. Interested readers are referred to Korenaga
[2004] for a complete description. Here I just explain
the main results and their geological implications.
Two models are shown for comparison: one with isochemical
mantle and the other with segregated crustal fragments.
The former serves as a reference, showing what would
be expected for the simplest setting.
Color indicates potential temperature,
yellow is hottest, and dark blue coldest. The model
extends down to the base of the whole mantle. The lower
mantle has 30 times higher viscosity than the upper
mantle, and there is also a moderate endothermic phase
change at 660 km. These numerical models are designed
to investigate the dynamic state of the mantle prior
to continental breakup.
Case A: Isochemical Model
Viscosity layering and the endothermic
phase change result in layered convection at least partially
and also temporally. A completely layered state is dynamically
unstable, and whole-mantle-scale flow periodically flushes
through the system. This is very similar in nature to
what is known as “mantle avalanche” in mantle
convection models, though the temperature variations
involved are much smaller here. Whenever large-scale
downwelling takes place, there is also corresponding
upwelling with similar strength. (Note that whole-mantle-scale
flow is much faster than upper-mantle-scale flow).
Figure 12: Case A. (a-d) Snapshots
of temperature and velocity fields. Velocity arrows
are normalized to maximum velocity, which is denoted
at every snapshot. Elapsed time shown here is measured
from the onset of convection, after which lithosphere
is thicker than ~100 km. (e) Vertical mass flux (VMF)
diagnostic with contour interval of 2.0. It can be seen
that most of the vertical mass flux is confined above
660 km for the first 150 Myr. (f) Maximum upwelling
(solid) and downwelling (dotted) velocities observed
in the model domain.
Case B: With Segregated Crust (green
dots)
Case B includes segregated crustal
fragments floating at 660 km (shown as green dots).
These crustal fragments are denser than the upper mantle,
but more buoyant than the lower mantle. Therefore, it
is very difficult to push them down, and downwelling
takes place preferentially away from these fragments.
This results in the fragments spreading out horizontally,
which makes them more easily penetrated by counter upwelling.
Counter upwelling is indeed strong enough to entrain
as much as 20-30% of the fragments (see bottom of the
figure). Interestingly, upwelling is also spatially
focused; it is not broad and diffuse.
Figure 13: Case B. (a-d) Snapshots
of temperature and velocity fields, as in the previous
figure. Tracers for crustal fragments are denoted by
green circles. (e) Vertical mass flux diagnostic. (f)
Maximum upwelling (solid) and downwelling velocities
(dotted). (g) Maximum fraction of crustal fragments
entrained into the upper mantle shallower than 410 km.
Concluding Remarks
My convection model is relatively
simple, i.e., a cooling whole-mantle system with segregated
crust accumulated at the 660-km discontinuity. Yet,
this incomplete state of mantle mixing can induce quite
complex dynamics, with profound implications for the
origin of anomalous magmatism. Voluminous breakup magmatism
may simply reflect compositional heterogeneity in the
upper mantle, not excess mantle temperature associated
with a deep thermal boundary layer. It is less problematic,
for example, to account for the proximity of the Iceland
and the Jan Mayen hotspots, which are only ~800 km apart,
by upper mantle heterogeneity than two closely-located
plumes rising from the depth of ~3000 km. The mobility
of the Iceland hotspot with respect to other Indo-Atlantic
hotspots [Norton, 2000] is also no more surprising
because counter-flow entrainment is not strongly anchored
in the mantle. In addition, the amount of crustal fragments
embedded in the mantle matrix is unlikely to be constant
over time, considering the random nature of entrainment,
so it is natural to expect time-varying melt productivity,
which we may observe today as the V-shaped Reykjanes
Ridge [Vogt, 1971].
Though my model may be best characterized
by the chemically heterogeneous upper mantle, it is
not strictly an upper-mantle model. The lower mantle
is also involved; counter upwelling is supplied from
the lower mantle. This may explain why the Iceland hotspot
has high 3He/4He ratios, which
are usually considered as a signature of relatively
undegassed deep mantle reservoir [e.g., Kurz et
al., 1982] (Editor's note: see also Helium
fundamentals page, however, for an upper-mantle
model for high-3He/4He), whereas
mantle tomography exhibits low velocity anomalies only
in the upper mantle. Sublithospheric mantle is likely
to be in a fully dynamic state on various spatial scales,
which is clearly seen even in the isochemical case.
Rifting above downwelling mantle may give rise to non-volcanic
rifted margins such as the Newfoundland Basin and the
Iberia margin.
Because entrained crustal fragments
start to melt deeper than surrounding mantle, melt-retention
buoyancy becomes important. This contrasts with the
melting of homogeneous mantle beneath normal mid-ocean
ridges, for which retention buoyancy is probably small
owing to the formation of a porous melt network. Thus,
a recent seismic study suggests buoyancy-driven mantle
upwelling beneath Iceland [Gaherty, 2001] is
consistent with the melting of chemically heterogeneous
mantle. Rapid lateral spreading in response to the basal
topography of lithosphere is also possible for upwelling
mantle with high melt-retention buoyancy; such dynamics
are not limited to plume-lithosphere interaction [Sleep,
1997]. Eventually, the surrounding mantle matrix starts
to melt at shallow depths (~60 km), and melt from the
entrained crust will escape into the porous network
developed in the matrix.
The thermal plume hypothesis assumes
that the mantle is homogeneous in terms of major-element
composition and attributes excess magmatism to elevated
mantle temperature only. This assumption is startling
in the light of the well-accepted fact that trace-element
and isotope heterogeneities exist in the mantle. Even
if a perfectly homogeneous mantle existed at some point,
it is not obvious how such homogeneity could be maintained
because plate tectonics continuously introduces heterogeneity
by chemical differentiation at mid-ocean ridges. Remixing
could well be incomplete sometime somewhere in a complex
convection system. Beyond the North Atlantic igneous
province, the number data available to distinguish between
the thermal and chemical origins is surprisingly small.
Speculation is fine and can be thought-provoking, but
we really need to acquire more new data to move on,
along with developing a more thorough understanding
of mantle dynamics.
|
References
- Allegre et al., 1977, Contrib. Miner. Petrol.,
60, 57-75.
- Coffin and Eldholm, 1994, Rev. Geophys.,
94, 7685-7729.
- Cordery et al., 1997, J. Geophys. Res., 102,
20179-20197.
- Gaherty, 2001, Science, 293, 1645-1647.
- Hanan and Graham, 1996, Science, 272, 991-995.
- Holbrook et al., 2001, Earth Planet. Sci.
Lett., 190, 251-266.
- Klein and Langmuir, 1987, J. Geophys. Res.,
92, 8089-8115.
- Karato, 1997, Phys. Earth Planet. Int., 99,
103-111.
- Korenaga et al., 2000, J. Geophys. Res.,
105, 21591-21614.
- Korenaga and Kelemen, 2000, Earth Planet.
Sci. Lett., 184, 251-268.
- Korenaga and Jordan, 2001, Geophys. J. Int.,
147, 639-659.
- Korenaga and Jordan, 2002, Geophys. J. Int.,
149, 179-189.
- Korenaga et al., 2002, J. Geophys. Res.,
107, 2178.
- Korenaga and Jordan, 2004, J. Geophys. Res.,
109, B01405.
- Korenaga, 2004, Earth Planet. Sci. Lett.,
218, 463-473.
- Kurz et al., 1982, Nature, 297, 43-47.
- Leitch and Davies, 2001, J. Geophys. Res.,
106, 2047-2059.
- Lesher, 2002, EOS Trans. AGU, 83, F1040.
- Marcano et al., 1999, J. Geodyn., 28, 75-95.
- Montelli et al., 2004, Science, 303, 338-343.
- Norton, 2000, in "The History and Dynamics
of Global Plate Motions", AGU, Washington, D.C.,
pp. 339-357.
- O'Hara, 1975, Nature, 253, 708-710.
- Ringwood and Irifune, 1988, Nature, 331,
131-136.
- Ritsema and Allen, 2003, Earth Planet. Sci.
Lett., 207, 1-12.
- Schilling, 1985, Nature, 314, 62-67.
- Sleep, 1997, J. Geophys. Res., 102, 10001-10012.
- Vogt, 1971, Earth Planet. Sci. Lett., 13,
153-160.
- Yasuda et al., 1994, J. Geophys. Res., 99,
9401-9414.
|
last updated 12th September,
2004 |
|