 |
|
The
authors of this page request feedback to help them mature
the ideas described herein.
|
Abstract
Ridge-crossing volcanic island chains
have not been satisfactorily explained before using
non-plume hypotheses and as such, ridge-crossing chains
are cited as evidence for relatively fixed deep-mantle
plumes. In this paper we describe two melt sources
whose interaction with ridges may produce age-progressive,
ridge-crossing volcanic seamount chains without invoking
a deep mantle source. We use both conceptual and finite-element
models to illustrate the viability of these mechanisms.
Introduction
Hypotheses for ridge-crossing
seamount chains have focused on the interaction
of mantle plumes with ridge segments (DePaolo
& Manga,
2003; Sleep 2002). A stationary
plume of hot mantle material rises to the
base of the lithosphere and creates a chain
of age-progressive seamounts. As a migrating
ridge approaches the plume, material rises
along the lithospheric gradient to the ridge
and chains of seamounts are created on both
sides of the ridge. Eventually the ridge moves
away from the plume and seamount production
on a single plate resumes (e.g., DePaolo
& Manga,
2003; Sleep 2002; Ribe
1996; Kincaid et al., 1996). This
results in the formation of a lopsided V-shaped
array of seamounts (Figure 1).
|
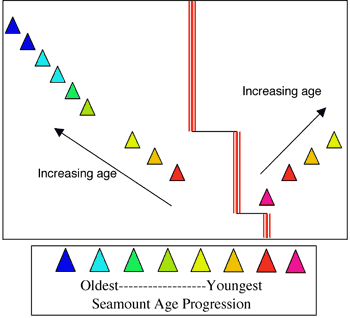
Figure
1: Cartoon of a ridge-crossing seamount chain
geometry in the Atlantic (after Sleep, 2002). |
Because current non-thermal models of
seamount formation are unable to explain this geometry,
(e.g. propagating cracks are presumed to
be unable to cross ridges), ridge-crossing seamount
chains have been cited as evidence for relatively
stationary plumes. However, we propose that when ridges
are viewed as active rather than passive features,
non-thermal ridge-crossing seamount chains are not
only viable, but also likely. In this paper we propose
an alternative model for ridge-crossing seamount chains
that does not invoke plumes but is still able to explain
the four primary elements:
-
a source of
melt,
-
a means of
bringing melt to the surface,
- a ridge-crossing seamount chain, and
-
an explanation
for the age progressive geometry of the chain.
Model
The source
of melt
We focus on two alternative sources
for the melt:
In the former case, the mantle
is heterogeneous and contains fertile streaks, some
of which are large and produce major outpourings of
magma when tapped, i.e. when the stress condition
in the overlying plate permits dikes and volcanoes
to form. In the latter case, the mantle is fairly
homogeneous but is at or near its melting point and
melt is created at any time pressure drops or when
the lithosphere extends and passive upwelling occurs
as a result. Elements of these scenarios have been
discussed previously by various authors, e.g.,
Meibom &
Anderson
(2003), Sleep (2002), Natland
& Dick
(2001), Niu et al. (2001), McNutt
& Bonneville (1999), Anderson (1998)
and Sleep (1997).
Transport
of melt to the surface
Melt is generated from fertile
mantle regions and mantle in a nascent melting condition
when it is decompressed. Decompression melting may
occur at and ahead of ridge-transform intersections
(RTIs) when slip along the transform is impeded (Beutel,
2005) This model for creating lithospheric extension
is suggested by the observation that many seamount
chains intersect or originate near RTIs, e.g.,
the Foundations and the St. Helena and Easter chains.
Finite-element models show that
large areas of extension develop when transform slip
is impeded (Figure 2a,b) (Beutel,
2005, in which a discussion of the possible causes
of impeded transform slip may be found; see also Ridge-transform
intersections page). If the mantle is already
partially molten, or if magma is ponded beneath the
plate, then the condition for dikng is simply that
the least compressive axis is horizontal. If the mantle
is entirely subsolidus, then decompression is required
to generate excess melt. This is expected to occur
in response to exension, such as occurs in the neighborhood
of RTIs. Seamount chains rather than linear ridges
are postulated to form as a result of periodic strengthening
and weakening of the transform fault due to changes
in the local stress state, which occur partially as
a result of the injection of magma into the crust.
We also postulate that the age difference between
a seamount and the underlying oceanic crust can vary
greatly depending on the offset of the transform and
the distance ahead of the RTI that the seamount is
emplaced.
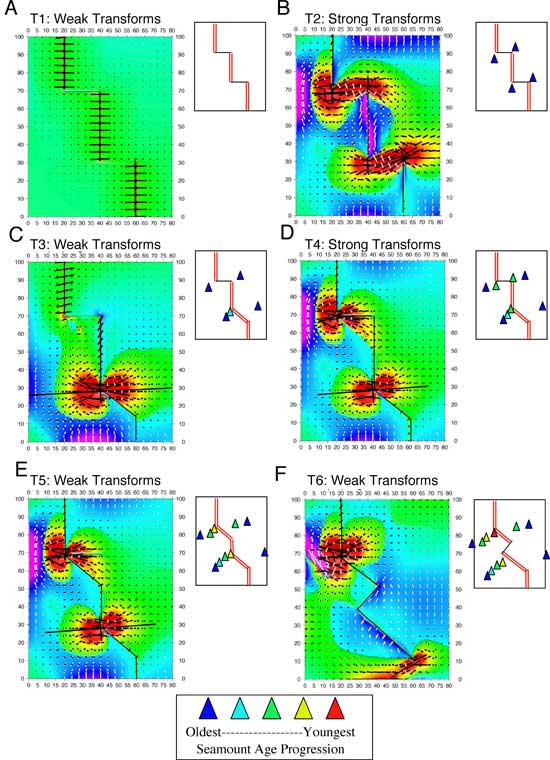
Figure 2: Results
of finite-element modeling of the nascent-melting
case. Panels show a series of 2D models of a reorganizing
ridge. Background colors indicate maximum stress intensity,
warmer colors indicate larger stresses, white and
black bars indicate maximum and minimum stress vectors
(white: compressional, black: extensional). Panels
A, C and E show the results for weak transforms (slip
not impeded), and panels B, D and F show strong transforms
(slip impeded). Note the increased extensional stress
at and ahead of the ridge-transform intersection when
slip is impeded. Adjacent to each panel is a cartoon
showing the seamounts that would be produced. Seamounts
are color-coded according to age, as shown in the
key at the bottom. These seamounts form time-progressive
chains. Panel F shows the switch from a dual seamount
chain on either side of the ridge to a single chain
on the western side of the ridge. Note the gap between
the chain on the east and the chain on the west. The
change in trend of the seamount chains on the western
plate reflects a relative, not absolute plate motion
change. Click here
for enlargement.
Ridge-crossing
seamount chains
Based on our understanding
of non-thermal melt sources and the transport of melt
to the surface we have developed two models by which
non-plume ridge-crossing seamount chains may be generated.
These models are based on the following assumptions:
-
most transforms
experience periods of decreased slip, due to welding
or tectonic forces,
-
when transform
slip is impeded, extension is concentrated at and
ahead of the RTI (Figure 2a,b),
-
if the mantle
is fertile or near its solidus, extension at an
RTI will result in the formation of a seamount,
and
-
ridges migrate
and reorganize.
Model 1 – A
reorganising ridge: Model 1 is illustrated in Figure
2. We examine a possible ridge-crossing geometry for
seamount chains formed during a ridge reorganization
over a near-solidus mantle. We assume that any large,
concentrated extension in the oceanic lithosphere will
result in the formation of a seamount. Finite-element
models of an evolving ridge were constructed to determine
the location of these extensional stresses and the resultant
seamount pattern that would emerge, during a ridge reorganization.
More than 12 initial time frames were constructed, and
the 6 most relevant are shown. Figure 2 presents the
finite-element model results as maximum stress type
by color (red is extensional and blue is compressional)
and maximum and minimum stress vectors, along with conceptual
models of seamount emplacement adjacent to the stress
maps. The exact model parameters are given in the appendix.
Stresses applied to the model consist chiefly of ridge-perpendicular
gravity forces applied to the whole of the plate.
-
Time 1: Three north-south-trending
ridge segments are connected by weak transforms.
The oceanic plate is under relatively little east-west
stress.
-
Time 2: A change
in plate motion direction results in NE-SW ridge-push
forces and the strengthening of both transform faults.
Large areas of extensional stress are concentrated
ahead of and at the RTIs. Seamounts are emplaced
in older crust ahead of the RTIs.
-
Time 3: In response
to changing plate motions a new NW-SE-oriented ridge
propagates between the southern two ridge segments,
eradicating the transform. The northern transform
is modeled as weak. Extension is concentrated where
the new NW-SE trending ridge intersects the middle
NS-trending ridge segment. Seamounts are emplaced
on the western plate only.
-
Time 4: Re-strengthening
of the northern transform results in extensional
stresses at and ahead of the RTIs there. New seamounts
are emplaced.
-
Time 5: A new NW-SE-trending
ridge segment propagates over the northern transform.
Large extensional stresses are concentrated where
the NS ridge segments intersect the NW-SE trending
segments, more seamounts are emplaced on the western
plate.
-
Time 6: A new, weak
transform forms between the NW-SE trending ridge
segments. Extension is still concentrated at the
intersection of the NS- and NW-SE-trending ridge
segments. Seamounts are emplaced on the western
plate at the intersection.
Seamount
Pattern: The resulting seamount pattern is a pair
of chains that age progressively away from the ridge
and appear to switch from emplacement on both sides
of the ridge to emplacement only the west side. This
gives the appearance of a ridge that overran a hotspot.
While no one specific
seamount chain is modeled in Figure 2, the ridge reorganization
modeled is similar to ridge reorganizations seen throughout
the Pacific plate. The high density of seamounts in
the Pacific compared with the Atlantic suggests that
the mantle beneath the Pacific is closer to its solidus
than Atlantic mantle.
Model 2 – A
stationary fertile region: In this conceptual model
we apply the finite-element results of extension at
or ahead of RTIs to a ridge migrating over a stationary
fertile mantle region. The assumption is that seamounts
will only be formed when there is strong extensional
stress above the fertile mantle region, the presumed
source of the extensional stress being impeded slip
on a transform fault.
Figure 3 is a schematic
diagram illustrating the modeling results separated
into discrete time periods. During the first four time
periods (T1 - T4) a fertile mantle region is intersected
by a series of RTIs as the ridge moves to the northwest.
This results in a single chain of seamounts on the western
plate, the spacing of which is determined by the production
rate at, and spacing of, the RTIs. The seamounts move
to the northwest relative to one-another but to the
west relative to the ridge. In time period T5 the ridge
overrides the fertile mantle region and chains of seamounts
on both sides of the ridge are created. Finally, the
ridge moves off the fertile mantle region and the chain
on the western plate is terminated. In time period T8
the fertile mantle region is intersected by an RTI on
the eastern side of the ridge and a new series of seamounts
is created, giving the impression that the seamount
chain has crossed the ridge.
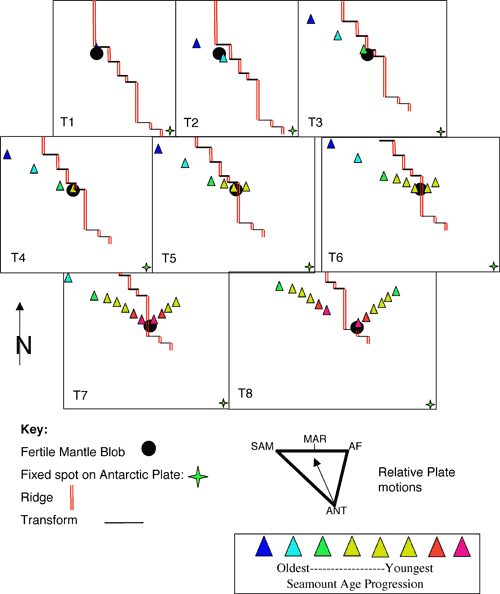
Figure
3: Time series for a conceptual model of a ridge and
a fertile mantle region migrating with respect to
a fixed point (crescent) on the eastern (African)
plate. In time periods T1-T4 impeded slip along the
transform increases extension over the fertile mantle
region, resulting in extension and volcanism, and
seamounts are emplaced on the western plate. During
time periods T5-T7 the fertile mantle region lies
beneath the ridge and seamounts are emplaced on both
plates. In time period T8 the ridge moves away from
the fertile mantle region, resulting in the formation
of seamounts on the eastern plate. Because the region
of fertility migrates south relative to the fixed
point on the eastern plate, a pair of time-progressive
NW- and NE-trending seamount chains forms.
Other
Locations
The model above can
account for the geometries of some Atlantic seamount
chains. However, the principles of RTI-generated seamount
chains may be applied to many other observed seamount
geometries. Short seamount chains may result from
small regions of mantle fertility and/or the migration
of a ridge away from the fertile region. Large areas
of melt and aseismic ridges may represent large regions
of fertility or mantle in a nascent melting state.
The more numerous seamounts and faster spreading rates
in the South Pacific indicate that different processes
may affect seamount formation there including strong
thermal contraction of the lithosphere, a plate close
to the tensile state, and/or numerous fertile patches
or proximity to the nascent melting state.
Large
igneous provinces (LIPs)
Plume-based models for
ridge-crossing-hotspots involve the separation of
postulated plume heads (LIPs) from the ends of their
tails (volcanic chains) by actively spreading ridges.
Most volcanic chains, however, do not start at a LIP
and most LIPs are not associated with a volcanic chain.
Perhaps the best documented case is the separation
of the Kerguelen Plateau from the Ninety-East Ridge
and the Rajmahal basalts (Weis et al., 2002;
Kent et al., 2002; Coffin et al.,
2002). In order to link the basaltic outpourings on
mainland India with the Kerguelen Plateau and Broken
Ridge a series of ridge jumps are postulated to have
occurred as the Kerguelan hotspot drifted slowly to
the south (Kent et al., 2002; Antretter
et al., 2002). Other models involve multiple
plumes (e.g., Coffin et al., 2002). In this
paper we highlight, as others have before us, the
links between LIPs, ridges and transform faults, whereby
many of the proposed splits between "plume heads"
and "plume tails" may be ridge-reorganizations
and transform fault interactions rather than ridges
crossing fixed thermal anomalies. The prevalence of
"coincidental" relationships between hotspot
features and tectonic features is thought-provoking.
These include:
-
the Ninetyeast ridge
lies is along an extensive offset of seafloor magnetic
anomalies – a fossil transform fault,
-
Réunion is
located on the intersection of an abandoned ridge
and a fracture zone,
-
Réunion and
Mauritius developed on Paleocene fossil spreading
centers and were transported away from each other
by a fracture zone that lies in between ( Hirn,
1993), and
-
the Ontong Java
plateau is thought to have been created at a triple
junction ( e.g., Korenaga,
2005).
Discussion
Seamount chains that apparently
cross ridges do not require a plume-based model, but
can be explained by ridge dynamics and mantle heterogeneities.
Our models demonstrate that volcanism can migrate from
one side of a ridge to the other when extensional stress
at and ahead of RTIs is considered. We further suggest
that the nature of the melt sources may also affect
seamount-chain geometry. Regions of fertility in the
mantle may have the ability to produce larger volumes
of magma than thermal anomalies of the magnitudes suggested
by observations (see also Thermal
pages). The recognition that hotspots move relative
to one another and relative to the geomagnetic reference
frame, led to the concept of drifting plumes, the predicted
consequences of which are not different from those of
a passive fertile heterogeneity. A stress-controlled
mechanism of magma release is, however, more able to
cause the rapid high-rate volcanism that builds LIPs,
and the rapid switching on and off of magmatism along
volcanic chains, than a thermal plume explanation. Furthermore,
the size of a fertile heterogeneity is not important
since it is stress, and the extension of the lithosphere,
that controls and localizes the volcanism.
The melting of fertile
patches of mantle requires no additional heat input
to explain even the largest volumes of melt produced
(Korenaga,
2005). The largest LIP on Earth is the Ontong-Java
Plateau (see also Ontong
Java pages). If the 20-km-thick crust there resulted
from draining an area three times larger than the plateau
itself (as a result of focusing at the apex of a triple
junction) and if 20% melting was involved, then only
a 30-km-thick section of the mantle may have been involved.
The normal mantle geotherm is usually close to or above
the solidus in the depth range ~30 to ~50 km. Clift
(2004) has shown that the subsidence patterns of many
oceanic plateaus attributed to plumes are consistent
with normal mantle temperatures and not the elevated
temperatures expected for plumes (see also Mantle
temperature under LIPs page). This is consistent
with the model we propose here, which attributes ridge-crossing
seamount chains and LIPs to the extraction of melt from
regions of fertility and does not invoke greatly elevated
temperature.
Conclusions
Many so-called hotspot
tracks lie along pre-existing fracture zones and transform
faults, or emerge from RTIs. This, along with the inability
of propagating cracks to cross active spreading ridges,
led us to explore mechanisms for the migration of stress
conditions as an explanation for the volcanism. A fertile
region in the mantle can also have similar effects to
a hotspot (plume). Finite-element models demonstrate
the viability of off-ridge extensional stress migration
associated with ridge-transform intersections (RTIs).
Combined with fertile mantle and/or mantle in the nascent
melting condition, such areas of extensional stress
may create ridge-crossing seamount chains without the
need to invoke a thermal anomaly for which there is
no independent evidence.
Many of the geochemical
arguments for “plume" compositional components
could apply equally well to passive compositional hetereogeneities.
Our models do not rule out a thermal plume origin for
ridge-crossing seamount chains, but they demonstrate
that such a model is non-unique. A passive mantle heterogeneity
is not expected to be relatively fixed. The bulk of
the upper mantle is probably moving, albeit much more
slowly than plates and ridges. However, a source sufficiently
deep, i.e. beneath the plate, is all that is required,
as in the original model of Wilson (1963). |
References
-
Anderson,
D.L., 1998, The scales of mantle convection, Tectonophysics,
284, 1-17, 1998.
-
Antretter,
M., B. Steinberger, F. Heider, and H. Soffel, Paleolatitudes
of the Kerguelen hotspot; new paleomagnetic results
and dynamic modeling: Earth and Planetary Science
Letters, 203, 635-650, 2002.
-
Beutel,
E.K., Stress Induced Seamount Formation at Ridge-Transform-Intersections,
in Foulger, G.R., Anderson, D.L., Natland, J.H.
and Presnall, D.C. eds., Plates, Plumes, and
Paradigms: Geological Society of America Special
Paper: 388, 581-593, 2005.
-
Clift,
P.D., Sedimentary evidence for moderate mantle temperature
anomalies associated with hotspot volcanism, , in
Foulger, G.R., Anderson, D.L., Natland, J.H. and
Presnall, D.C. eds., Plates, Plumes, and Paradigms:
Geological Society of America Special Paper 388,
279-287, 2005.
-
Coffin, M.F.,
M.S. Pringle, R.A. Duncan, T.P. Gladczenko, M. Storey,
R.D. Mueller, and L.A. Gahagan, Kerguelen hotspot
magma output since 130 Ma: Journal of Petrology,
43, 1121-1139, 2002.
-
-
Gobat, J.
I. and Atkinson, D. C., FElt: User's Guide and
Reference Manual: San Diego, University of
California, San Diego, pp 234, 1996.
-
-
Kent, R.W.,
M.S. Pringle, R.D. Mueller, A.D. Saunders, and N.C.
Ghose, 40Ar/39Ar geochronology
of the Rajmahal basalts, India, and their relationship
to the Kerguelen Plateau: Journal of Petrology,
43, 1141-1153, 2002.
-
-
Kincaid, C.,
Schilling, J.G., Gable, C., The dynamics of off-axis
plume-ridge interaction in the uppermost mantle:
Earth and Planetary Science Letters, 137,
29-43, 1996.
-
-
McNutt, M.,
and A. Bonneville, A shallow, chemical origin for
the Marquesas Swell: Geochemistry, Geophysics,
Geosystems, 1, 17, 1999.
-
-
Niu, Y., D.
Bideau, R. Hekinian, and R. Batiza, Mantle compositional
control on the extent of mantle melting, crust production,
gravity anomaly, ridge morphology, and ridge segmentation;
a case study at the Mid-Atlantic Ridge 33-35 degrees
N: Earth and Planetary Science Letters,
186, 383-399, 2001.
-
Ribe, N.M.,
The dynamics of plume-ridge interaction 2. Off-ridge
plumes, Journal of Geophysical Research,
101, 16,195-16,204, 1996.
-
Richardson,
R.M., Solomon, S.C., Sleep, N.H., Tectonic stress
in the plates: Reviews in Geophysical Space
Physics, 17, 981-1017, 1979.
-
Sleep, N.H.,
Lateral flow and ponding of starting plume material:
Journal of Geophysical Research, 102,
10,001-10,012, 1997.
-
Sleep, N.H.,
Local lithospheric relief associated with fracture
zones and ponded plume material: Geochemistry,
Geophysics, Geosystems, 3,
17, 2002.
-
Weis, D.,
F.A. Frey, R. Schlich, M. Schaming, R. Montigny,
D. Damasceno, N. Mattielli, K.E. Nicolaysen, and
J.S. Scoates, Trace of the Kerguelen mantle plume;
evidence from seamounts between the Kerguelen Archipelago
and Heard Island, Indian Ocean: Geochemistry,
Geophysics, Geosystems, 3,
16, 2002.
-
Wilson, J.T., A possible origin of the Hawaiian Islands, Can. J.
Phys., 41, 863-870, 1963.
|
Appendix:
Finite Element Model Parameters and Geometry
Model Type:
Two-dimensional plane strain elastic finite element
model, program by Gobat & Atkinson (1996).
Model Parameters:
Applied Forces:
Applied forces are ridge-perpendicular and applied to
the whole of the plate based on the modeled age of the
crust. As new ridges are propagated to the southwest
from the N-S trending ridges, new forces perpendicular
to the now NW-SE trending ridges are applied. The basic
applied forces are as follows:
Age
(Myr) |
Force
(N/m) |
|
|
0 |
2e+12 |
10 |
1.23e+11 |
20 |
9.17e+10 |
30 |
7.1e+9 |
40 |
5.1e+8 |
Strength:
The model consists of material of two end-member strengths,
weak and strong. The strong material is 3 orders of
magnitude stronger than the weak material. This is based
on the strong decoupling expected between ridge material
(weak) and oceanic crustal material (strong). Others
(Richardson et al., 1979) have demonstrated
that the exact ratio is not important as long as it
is > 1. Because this model was testing end-member
conditions the transform was alternately modeled as
both weak and strong.
Boundary Conditions:
The model is fixed in space along its eastern edge.
Numerous configurations were tested to determine the
configuration with the least edge effects. By fixing
the model on its eastern edge we allow it to move as
a whole while still providing a baseline to move against.
This is similar to modeling the apparent movement of
the entire South Atlantic basin away from a pinned African
plate. |
last
updated 21st November, 2005 |
|
|