 |
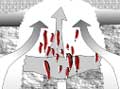 |
Basaltic
magmatism influenced by high-pressure basaltic lithologies
stored in the upper mantle |
|
Michele
Lustrino
Dipartimento di Scienze della
Terra, Università degli Studi di Roma La Sapienza,
P.le A. Moro, 5, 00185 Rome, Italy
and
Istituto di Geologia Ambientale e Geoingegneria (IGAG)
– CNR, P.le A. Moro, 5, 00185 Rome, Italy
michele.lustrino@uniroma1.it
|
Abstract
During the last decade,
the role of garnet clinopyroxenites (and, more generally,
basaltic compositions metamorphosed to high-density
assemblages in the mantle) in basaltic petrogenesis
has been the subject of several detailed investigations.
Experimental studies and geochemical/petrological modeling
suggest that pyroxenitic in sensu lato material
is a potential source component in basaltic magmatism
in general (e.g., Allégre & Turcotte,
1986; Meibom
& Anderson,
2003; Anderson,
2005). For example, recycling of former basaltic
material (in the form of garnet-clinopyroxenites, granulites,
eclogites, websterites, olivine websterites) has been
proposed to explain variability in MORB (Mid-Ocean Ridge
Basalt) geochemistry (e.g., Hirschmann & Stolper,
1996; Eiler et al., 2000), as well as in HiMu-OIB
(High Mu Oceanic Island Basalts, where Mu = μ
= 238U/204Pb) lavas (e.g.,
Kogiso et al., 2003; Sobolev et al., 2005),
both continental and oceanic EMI-like (Enriched Mantle
type 1) basalts (e.g., Lustrino
et al.,
2000; Lustrino
& Dallai,
2003; Escrig et al., 2004; Lustrino,
2005a,
2005b), subduction-related magmas (e.g., Schiano
et al., 2000), intra-plate igneous rocks (e.g.,
Gao et al., 2004; Zandt et al., 2004)
and continental flood basalts (CFBs; Cordery et
al., 1997; Yaxley, 2000).
Here I show that the delamination
and detachment of lower continental crust may be able
to bring crustal lithologies down to mantle depths.
The interaction of partial melts derived from these
lithologies (metamorphosed to pyroxenitic/granulitic/eclogitic
assemblages) with ambient mantle can explain some geochemical
features of volumetrically insignificant though petrologically
important magmas such as EMI-like basalts. Interaction
of “normal mantle” (but what is “normal
mantle”?) with lower-crust-derived partial melts
has been proposed, amongst others suggestions, to explain
the peculiar composition of the Plio-Quaternary volcanic
rocks of Sardinia, Italy (Lustrino et al.,
2000,
2005). In particular, the geochemical characteristics
of Sardinian rocks (high SiO2, low CaO and
CaO/Al2O3, relatively high Ni,
relatively low HFSE, low HREE, high Ba/Nb and La/Nb,
slightly high 87Sr/86Sr, unradiogenic
143Nd/144Nd and 206Pb/204Pb
ratios) have been linked to derivation from an orthopyroxene-rich
mantle source. The origin of this enrichment in orthopyroxene
is thought to be a consequence of reaction of the original
lithospheric mantle with SiO2-rich partial
melts derived from delaminated and detached ancient
lower continental crust.
Introduction
The geochemistry of igneous
rocks has often been considered an infallible tool to
explain the composition of Earth’s mantle. Major
elements, trace elements and the isotope ratios of undifferentiated
magmas (i.e. melts directly derived by partial melting
of the mantle without modification related to fractional
crystallization) reflect the composition of their sources.
Moreover, the geochemical characteristics of primitive
igneous rocks have often been used to infer the geodynamic
setting of formation or, in a more speculative way,
the thermal and rheological structure of the underlying
mantle (e.g., the presence of mantle plumes).
The composition of igneous rocks is the net effect of
many complex factors including interconnection of liquids
formed at various partial melting degrees at various
depths in equilibrium with different restitic mineralogies,
heterogeneous composition of mantle sources (i.e., presence
of pyroxenitic slices in a peridotitic medium; e.g.,
Liu et al., 2005) and chromatographic reactions
between rising partial melts and ambient mantle (e.g.,
Kogiso et al., 2004).
The result is that the
geochemical message carried by primitive igneous rocks
is not easily decipherable because of the many variables
required to model the system from the formation of the
first drop of melt to the solidification of the last
liquid. In this sense, proposing the existence of huge
structures in the Earth’s mantle (e.g.,
mantle plumes with roots anchored in the deep mantle)
only on the basis of geochemical argumentations is dangerous.
However, it should also be noted that petrology cannot
exclude the existence of such thermal anomalies on the
basis of geochemical data alone.
Geochemical
constraints
During the last two decades
some basic geochemical and petrological concepts have
been reconsidered. For example, the concept that the
source of MORB is homogeneous and depleted contrasts
with new data (e.g., Hofmann, 2003; Workman
& Hart, 2005) that are reconcilable only with
heterogeneous sources (i.e., pyroxenitic slices dispersed
in a depleted matrix; Allegre & Turcotte,
1986; Meibom
& Anderson,
2003; Anderson,
2005). Also other types of magmatism (e.g.,
related to OIB) have revealed a very wide range of chemical
compositions. Several acronyms have become part of geochemical
jargon (DMM, HiMu, EMI, EMII, FoZo, PHeM, C, PreMa and
so on) implying the existence of geochemical mantle
end-members not immediately associable with physical
entities (i.e., volumes in the mantle; Zindler &
Hart, 1986; Hofmann, 1997, 2003, Lustrino,
2001; Lustrino
& Dallai,
2003; Workman et al., 2004; Stracke
et al., 2005). For those not skilled in igneous
geochemistry these acronyms may lead to confusion. To
clarify matters, it may be said that most of such acronyms
have been invoked to explain the most extreme isotopic
compositions (mostly based on Sr-Nd-Pb isotope systematics,
plus a few constraints based on He-Os-Hf-O isotopes)
of basalts emplaced in oceanic settings (i.e., away
from places where the continental lithosphere can act
as potential contaminant). Trace- and major elements
are much more variable (e.g., Lustrino
& Dallai,
2003) than originally supposed (e.g., Weaver,
1991) and large overlaps may exist between single end-members.
To complicate the situation still further, the isotopic
composition of some end-members as proposed in literature
is continuously changing (e.g., the isotopic
definition of FoZo has changed four times during the
last 13 years; Stracke et al., 2005) and in
some cases it is impossible to propose a fixed isotopic
composition (e.g., the 207Pb/204Pb
isotopic ratios of EMI; Mahoney et al., 1996;
Lustrino
& Dallai,
2003).
What may be said with
certainty is that virtually all the geochemical end-members
(as revealed by the composition of primitive or only
slightly differentiated basalts) can be explained by
an origin from mantle sources that have experienced
interaction with crustal material introduced at depth.
Another key point is that geochemistry cannot be considered
as a proof for or against the existence of mantle plumes
coming from deep reservoirs (e.g., Lustrino,
2005a). Detailed study of the geochemical characteristics
of primitive igneous rocks has inspired several petrological
models that can explain isotopic and trace element characteristics
without invoking deep and isolated reservoirs (e.g.,
Foulger et al.,
2005 and references therein; Lustrino,
2001, 2005a;
Anderson,
2005; Stracke et al., 2005).
Mantle
plumes
Recently the classical
model of a mantle plume, considered to be a thermal
anomaly coming from a deep reservoir, has been subject
to strong criticism (e.g., Sheth,
1999; Lustrino,
2001, 2005; Foulger
et al., 2005,
and references therein). The concept of the mantle plume
has been considered suitable to explain the production
of the huge volumes of magma associated with CFBs and
Large Igneous Provinces (LIPs). Mantle plumes have also
been proposed to explain magmatic activity away from
active subduction systems and passive margins. At the
heart of the concept of the mantle plume is that it
is associated with a thermal anomaly coming from the
deep mantle.
The fundamental objection
to the classical hypothesis of the mantle plume, proposed
to explain both high- and low-volume magmatism in both
in continental and oceanic settings, is that the observed
anomaly is chemical rather than thermal. High melt productivity
from mantle sources can be obtained at “normal”
temperatures if basaltic material (metamorphosed to
high-density assemblages), or partial melt from basaltic
material, is interbedded in “normal” peridotite
(e.g., Cordery et al., 1997; Yaxley,
2000; Meibom
& Anderson,
2003; Anderson,
2005). Basaltic material (metamorphosed to garnet-clinopyroxenitic,
granulitic or eclogitic assemblages, depending on the
starting composition) is characterized by solidus temperatures
about 100°C lower than peridotite at the same pressure.
Most of the basaltic material
in the mantle is probably introduced at subduction zones.
However, another mechanism able to transport shallow
basaltic material to greater depth in the mantle is
the delamination and detachment of lower continental
crust (see Lustrino,
2005a, for a detailed discussion).
The lower crust and mantle
portions of continental lithosphere exert strong structural
and chemical controls on basaltic magmatism. Apart from
the obvious controls of subduction on magmatic geochemical
budgets and the role of buoyancy in trapping basaltic
magmas, I advance an alternative model that a combination
of lower crust and lithospheric mantle controls the
composition of both continental and oceanic intra-plate
magmatism, and magmatism associated with continental
break-up, particularly basalts with EMI affinity.
I propose a model that
posits a role for lower-crustal delamination and detachment
in the upper-mantle geochemical budget. This model can
explain the geochemical signature of volumetrically
insignificant, but petrologically important, EMI-like
magmas, and is an alternative to crustal recycling in
subduction zones.
Lower
crustal delamination and detachment
The model is based on
the development of gravitative instability following
continent-continent collision along mobile belts (Figure
1). Continent-continent collisions force lower crustal
rocks (with their original basaltic/gabbroic composition)
to higher pressures and temperatures. Under such conditions,
the lithospheric keel becomes gravitationally unstable
and may detach, sinking into the asthenospheric mantle
[Ed: see also other Lithospheric
thinning pages]. Metamorphic reactions occurring
in the lower continental crust as a consequence of continent-continent
collision can lead to density increases, with densities
rising to up to 3.8 g/cm3 with the appearance
of garnet in the metamorphic assemblage (basalt –
garnet – clinopyroxenite/eclogite). This may lead
to gravitative instability of the overthickened lithospheric
keel (the lower crust + lithospheric mantle) which may
detach from the uppermost lithosphere and sink into
the upper mantle. The volume formerly occupied by the
lithospheric keel is replaced by asthenosphere melts
(transformed into new lower crust) and asthenospheric
restite (which is transformed to lithospheric mantle,
i.e., the mechanical boundary layer, by cooling from
above). The lower crust, sinking to great depths, may
partially melt, yielding liquids of dacitic/rhyolitic
composition that react with the newly formed lithospheric
mantle, forming orthopyroxene-rich layers. After freezing,
such metasomes may also be reactivated several million
years after lithospheric delamination occurred (Lustrino,
2005a). The metasomatized lithosphere may thereby
acquire strong crustal geochemical imprints as commonly
observed in CFB (Continental Flood Basalts; e.g.,
Peate et al., 1999), as well as in continental
(e.g., Lustrino et al., 2000,
2005) and oceanic (e.g., Mahoney et al., 1996;
Borisova et al., 2001; Frey et al.,
2002) intra-plate basalts, and, rarely, mid-ocean ridge
magmas (e.g., Kamenetsky et al., 2001).
|
Figure 1: (A)
Initial situation with thin oceanic lithosphere
between thick continental plates. The continental
lithosphere is divided according to rheological
behavior: 1) brittle SiO2-rich upper continental
crust; 2) ductile mafic lower crust; 3) lithospheric
mantle, reaching an average depth of about 80
km, the crustal portion accounting for less than
half.
(B) Under compressive
stress, the oceanic lithosphere (colder and denser
than continental lithosphere) is subducted beneath
one of the two continental plates, with relatively
little deformation of the lower continental crust.
(C) Continent-continent
collision. The oceanic lithosphere has been completely
subducted.
(D) The upper
continental crust is piled up and thrusted, leading
to thickening of the entire lithosphere, including
the lower continental crust. Depending on the
extent of pressure increase, the original lower
crustal basaltic/gabbroic paragenesis may be transformed
to amphibolite (P ≤1 GPa) or to granulite/eclogite/garnet
clinopyroxenite assemblages at higher pressures
(~2-3 GPa; e.g., Wolf & Wyllie, 1994; Rapp
& Watson, 1995; Jull & Kelemen, 2001).
The net effect is a density increase to up to
3.5 g/cm3 (e.g., Wolf & Wyllie,
1993). These metamorphic reactions are not strictly
isochemical. During the formation of new phases,
Rb and U are preferentially concentrated in the
melt compared to Sr and Pb, respectively, while
Sm and Nd are not strongly fractionated. Restites
are therefore characterized by low to very low
Rb/Sr and U/Pb and relatively unchanged (low)
Sm/Nd ratios. The restite eclogite/garnet-clinopyroxenite
thus evolves with low time-integrated 87Sr/86Sr,
204Pb/206Pb and 143Nd/144Nd.
(E) The density
increase leads to gravitative instability of the
overthickened lithospheric keel. In particular,
dense garnet-rich lower-crustal restite causes
detachment of the lithospheric mantle from the
higher lithosphere levels and it sinks into the
asthenosphere. The high densities of average lower
crust contrast significantly with those of the
lithospheric mantle and asthenosphere, and there
is a strong tendency for the overthickened lithospheric
keel to sink.
(F) Detail of
(E). During sinking, the lower crust is likely
to undergo partial melting producing liquids of
TTG (Tonalitic, Trondhjemitic, Granitic) and adakitic
affinity (e.g., Springer & Seck, 1997; Defant
& Kepezhinskas, 2001; Zegers & van Keken,
2001; Xu et al., 2002). During lithospheric detachment,
new asthenospheric mantle replaces the region
vacated by delaminated lithospheric mantle and
lower crust. According to this model, the asthenosphere
accretes to the remaining lithosphere and becomes
transformed to lithosphere on cooling. Isostatic
uplift and formation of intermontane troughs accompany
delamination as a consequence of upward impingement
of hot, buoyant asthenosphere. Partial melts of
the asthenospheric megalith underplate the remaining
lithosphere to form the new lower crust and basaltic
magmatism at surface. Lithospheric mantle formed
from former asthenosphere is metasomatized by
SiO2-rich melts derived from the coeval
sinking of lower crust. Such highly reactive melts
would form orthopyroxene-rich zones, still peridotitic
in composition, and will therefore be able to
yield SiO2-undersaturated melts at
relatively high pressures (for more details see
Yaxley, 2000).
(G) After delamination
of the lower crust and lithospheric mantle, asthenospheric
counter flow, contemporaneous partial melting
of the lower crust and mechanical accretion of
the asthenosphere to the remaining lithosphere,
the new lithospheric mantle comprises variably
depleted peridotite, heterogeneously metasomatized,
with orthopyroxene-rich (lherzolite, olivine-pyroxenite,
websterite) lithologies. This mantle source may
then remain unsampled for several m.y. During
this period, lithospheric mantle metasomes evolve
with peculiar crustal isotopic features, i.e.:
1) elemental Sr originally present in plagioclase
is transferred to metasomatic melts during lower-crustal
partial melting; 2) the presence of residual garnet
in sinking lower crust produces partial melts
with strongly fractionated LREE/HREE evolving
with very low 143Nd/144Nd
isotopic ratios, and; 3) low m (m = 238U/204Pb)
crustal partial melts evolve with low 206Pb/204Pb
isotopic ratios.
(H) Metasomatized
lithospheric mantle may be reactivated several
m.y. after lower crustal delamination occurred.
Partial melts of such regions are likely to inherit
lower-crust-like metasomatic attributes, characterized
by typical EMI-like geochemical features (e.g.,
low radiogenic Pb ratios (206Pb/204Pb<
17), slightly radiogenic Sr isotopes (87Sr/86Sr
~0.706) unradiogenic Nd (143Nd/144Nd
~0.5121), unradiogenic Hf (176Hf/177Hf
~0.2826), slightly radiogenic Os (187Os/188Os
~0.135-0.145); Lustrino
& Dallai, 2003). Relative mantle-normalized
Ba, Pb, Eu or Sr anomalies and variation of Ba/Nb
ratios (3.5-47.4), Ce/Pb (1.2-24.6), Nb/U (10.5-71.8),
Sr/Nd (6.2-36.4) and Eu/Eu* (0.83-1.25) ratios
in EMI-type basalts (Lustrino
& Dallai, 2003) reflect the effects of
1) a lower-crust starting composition, 2) metamorphic
paragenesis and PT parameters conditioning lower-crust
partial melting, 3) metasomatic reactions between
SiO2-rich melts and peridotite, 4)
a cratonization style of asthenosphere, 5) partial
melting of newly-accreted lithospheric mantle,
and 6) fractional crystallization (coupled to
potential crustal assimilation) of lithospheric
melts.
|
Summary
Sources in the lowermost
mantle and anomalously high temperatures are not necessary
to explain the geochemistry of OIB and CFB magmas or
to achieve the requisite large melt fractions. An alternative
model is suggested by the presence of lower crustal
metasomatic signatures and related isotopic growth prior
to partial melting. Crustal material (either continental
lower crust or subducted oceanic slab) stored in the
upper mantle or the mantle transition zone is predisposed
to melting at ambient mantle temperatures.
Both the crustal and ultramafic
parts of the lithosphere may sink when cold, but become
buoyant when heated to ambient mantle temperatures.
The sinking of subducted lithospheric mantle may be
arrested at the 660 km discontinuity, where crustal
components begin to melt. Because of their buoyancy,
these melts trigger plume-like upwellings without the
need for lower mantle convective upwelling. The presence
of lithospheric material in the upper mantle can also
explain the isotopic features of both CFB and their
oceanic counterparts.
In conclusion, the role
of sinking lower crust and lithospheric mantle (and
of basaltic material recycled back into the mantle in
general) is critical to explaining the geochemical attributes
in CFB, OIB and LIP magmas. Delamination and detachment
of lower crust and lithospheric mantle are supported
by geophysical, geological, geochemical and petrological
considerations.
Acknowlegments
I warmly thank Gill Foulger
for her enthusiastic approach to the problem of the
chemical composition and the structure of upper mantle.
Her energy has been a continuous stimulus for my recent
studies.
|
References
-
Allegre J.-C.,
Turcotte D.L., 1986. Implications of a two-component
marble-cake mantle. Nature, 323,
123-127.
-
-
Borisova A.Yu.,
Belyatsky B.V., Portnyagin M.V., Sushchevskaya N.M.,
2001. Petrogenesis of olivine-phyric basalts from
the Aphanasey Nikitin Rise: evidence for contamination
by cratonic lower continental crust. J. Petrol.,
42, 277-319.
-
Cordery M.J.,
Davies G.F., Campbell I.H., 1997. Genesis of flood
basalts from eclogite-bearing mantle plumes.
J. Geophys. Res., 102, 20179-20197.
-
Defant M.J.,
Kepezhinskas P., 2001. Evidences suggests slab melting
in arc magmas. Eos, Trans. Am. Geophys. Un.,
82, 65-69.
-
Eiler J.M.,
Schiano P., Kitchen N. Stolper E.M., 2000. Oxygen-isotope
evidence for recycled crust in the sources of mid-ocean-ridge
basalts. Nature, 403,
530-534.
-
Escrig S.,
Capmas F., Dupré B., Allégre C.J.,
2004. Osmium isotopic constraints on the nature
of the DUPAL anomaly from Indian mid-ocean-ridge
basalts. Nature, 43, 59-63.
-
-
Frey F.A.,
Weis D., Borisova A.Yu., Xu G., 2002. Involvement
of continental crust in the formation of the Cretaceous
Kerguelen Plateau: new perspectives from ODP Leg
120 sites. J. Petrol., 43,
1207-1239.
-
Gao S., Rudnick
R.L., Yuan H.-L., Liu X.-M., Liu Y.-S., Xu W.-L.,
Ling W.-L., Ayers J., Wang X.-C., Wang Q.-H., 2004.
Recycling lower continental crust in the north China
Craton. Nature, 432, 892-897.
-
Hirschmann
M.M., Stolper E.M., 1996. A possible role for garnet
pyroxenite in the origin of the "garnet signature"
in MORB. Contrib. Mineral. Petrol., 124,
185-208.
-
Hofmann A.W.,
1997. Mantle geochemistry: the message from oceanic
volcanism. Nature, 385,
219-229.
-
Hofmann A.W.,
2003. Sampling mantle heterogeneity trough oceanic
basalts: isotopes and trace elements. In: R.W. Carlson,
H.D. Holland and K.K. Turekian (Ed.) Treatise
on geochemistry Vol. 2: The mantle and core,
61-101.
-
Kamenetsky
V.S., Maas R., Sushchevskaya N.M., Norman M.D.,
Cartwright I., Peyve A.A., 2001. Remnants of Gondwanan
continental lithosphere in oceanic upper mantle:
evidence from the South Atlantic Ridge. Geology,
29, 243-246.
-
Kogiso T.,
Hirschmann M.M., Frost D.J., 2003. High-pressure
partial melting of garnet pyroxenite: possible mafic
lithologies in the source of ocean island basalts.
Earth Planet. Sci. Lett., 216,
603-617.
-
Kogiso T.,
Hirschmann M.M., Petermann M., 2004. High-pressure
partial melting of mafic lithologies in the mantle.
J. Petrol., 45, 2407-2422.
-
Jull M., Kelemen
P.B., 2001. On the conditions for lower crustal
convective instability. J. Geophys. Res.,
106, 6423-6446.
-
Liu Y., Gao
S., Aeolus Lee C.-T., Hu S., Liu X., Yan H., 2005.
Melt-peridotite interactions: links between garnet
pyroxenite and high-Mg# signature of continental
crust. Earth Planet. Sci. Lett., 234,
39-57.
-
-
-
Lustrino M.,
2005b. Pyroxenites everywhere. Comment on “High-pressure
melting experiments on garnet clinopyroxenite and
the alkalic to tholeiitic transition in ocean-island
basalts” by Keshav et al. [Earth Planet. Sci.
Lett. 223 (2004) 365-379]. Submitted to Earth
Planet. Sci. Lett.
-
-
-
Lustrino M.,
Melluso L., Morra V., 2005. The uniqueness of Plio-Quaternary
volcanic rocks of Sardinia in the circum-Mediterranean
Cenozoic Igneous Province. Submitted to Geol.
Soc. Am. Spec. Paper, L. Beccaluva, G. Bianchini
and M. Wilson (Eds.).
-
Mahoney J.J.,
White W.M., Upton B.G.J., Neal C.R., Scrutton R.A.,
1996. Beyond EM-1: lavas from Afanasy-Nikitin Rise
and the Crozet Archipelago, Indian Ocean. Geology,
24, 615-618.
-
-
Rapp R.P.,
Watson E.B., 1995. Dehydration melting of metabasalt
at 8-32 kbar: implications for continental growth
and crust-mantle recycling. J. Petrol.,
36, 891-931.
-
-
Sobolev A.V.,
Hofmann A.W., Sobolev S.V., Nikogosian I.K., 2005.
An olivine-free mantle source of Hawaiian shield
basalts. Nature, 434,
590-597.
-
Springer W.,
Seck H.A., 1997. Partial fusion of basic granulites
at 5 to 15 kbar: implications for the origin of
TTG magmas. Contrib. Mineral. Petrol.,
127, 30-45 .
-
Stracke A.,
Hofmann A.W., Hart S.R., 2005. FOZO, HIMU, and the
rest of the mantle zoo. Geochem. Geophys. Geosyst.,
6, doi:10.1029/2004gc000824.
-
Weaver B.L.,
1991. Trace element evidence for the origin of ocean-island
basalts. Geology, 19,
123-126.
-
Wolf M.B.,
Wyllie P.J., 1993. Garnet growth during amphibolite
anatexis: implications of a garnetiferous restite.
J. Geol., 101, 357-373.
-
Wolf M.B.,
Wyllie P.J., 1994. Dehydration-melting of amphibolite
at 10 kbar: the effects of temperature and time.
Contrib. Mineral. Petrol., 115,
369-383.
-
Workman R.K.,
Hart S.R., 2005. Major and trace element composition
of the depleted MORB mantle (DMM). Earth Planet.
Sci. Lett., 231, 53-72.
-
Workman R.K.,
Hart S.R., Jackson M., Regelous M., Farley K.A.,
Blusztajn J., Kurz M., Staudigel H., 2004. Recycled
metasomatized lithosphere as the origin of the Enriched
Mantle II (EM2) end-member: evidence from the Samoa
volcanic chain. Geochem. Geophys. Geosyst.,
doi:10.1029/2003gc000623.
-
Xu J.-F.,
Shinjo R., Defant M.J., Wang Q., Rapp R.P., 2002.
Origin of Mesozoic adakitic intrusive rocks in the
Ningzhen area of East China: partial melting of
delaminated lower continental crust? Geology,
30, 1111-1114.
-
Yaxley G.M.,
2000. Experimental study of the phase and melting
relations of homogeneous basalt + peridotite mixtures
and implications for the petrogenesis of flood basalts.
Contrib. Mineral. Petrol., 139,
326-338.
-
Zandt G.,
Gilbert H., Owens T.J., Ducea M., Saleeby J., Jones
C.H., 2004. Active foundering of a continental arc
root beneath the southern Sierra Nevada in California.
Nature, 431, 41-46.
-
Zegers T.E.,
van Keken P.E., 2001. Middle Archean continent formation
by crustal delamination. Geology, 29,
1083-1086.
-
Zindler A.,
Hart S., 1986. Chemical Geodynamics. Ann. Rev.
Earth Planet. Sci., 14, 493-571.
|
last updated 8th
July, 2005 |
|
|